-
Enhancing Solanum lycopersicum Resilience: Bacterial Cellulose Alleviates Low Irrigation Stress and Boosts Nutrient Uptake
-
Diclofenac Interacts with Photosynthetic Apparatus: Isolated Spinach Chloroplasts and Thylakoids as a Model System
-
Diplodia seriata Isolated from Declining Olive Trees in Salento (Apulia, Italy): Pathogenicity Trials Give a Glimpse That It Is More Virulent to Drought-Stressed Olive Trees and in a Warmth-Conditioned Environment
-
Microbial Biocontrol Agents and Natural Products Act as Salt Stress Mitigators in Lactuca sativa L.
-
Azolla as a Safe Food: Suppression of Cyanotoxin-Related Genes and Cyanotoxin Production in Its Symbiont, Nostoc azollae
Journal Description
Plants
Plants
is an international, scientific, peer-reviewed, open access journal on plant science published semimonthly online by MDPI. The Australian Society of Plant Scientists (ASPS), the Spanish Phytopathological Society (SEF), the Spanish Society of Plant Biology (SEBP), the Spanish Society of Horticultural Sciences (SECH) and the Italian Society of Phytotherapy (S.I.Fit.) are affiliated with Plants and their members receive a discount on the article processing charges.
- Open Access— free for readers, with article processing charges (APC) paid by authors or their institutions.
- High Visibility: indexed within Scopus, SCIE (Web of Science), PubMed, PMC, PubAg, AGRIS, CAPlus / SciFinder, and other databases.
- Journal Rank: JCR - Q1 (Plant Sciences) / CiteScore - Q1 (Ecology, Evolution, Behavior and Systematics)
- Rapid Publication: manuscripts are peer-reviewed and a first decision is provided to authors approximately 18.2 days after submission; acceptance to publication is undertaken in 2.8 days (median values for papers published in this journal in the first half of 2024).
- Recognition of Reviewers: reviewers who provide timely, thorough peer-review reports receive vouchers entitling them to a discount on the APC of their next publication in any MDPI journal, in appreciation of the work done.
Impact Factor:
4.0 (2023);
5-Year Impact Factor:
4.4 (2023)
Latest Articles
Effects of Diverse Crop Rotation Sequences on Rice Growth, Yield, and Soil Properties: A Field Study in Gewu Station
Plants 2024, 13(23), 3273; https://doi.org/10.3390/plants13233273 - 21 Nov 2024
Abstract
This long-term field study conducted in Yancheng, China, evaluated the effects of diverse crop rotation sequences on rice growth, yield, and soil properties. Six rotation treatments were implemented from 2016 to 2023 as follows: rice–wheat (control), rice––rape, rice–hairy vetch, rice–barley, rice–faba bean, and
[...] Read more.
This long-term field study conducted in Yancheng, China, evaluated the effects of diverse crop rotation sequences on rice growth, yield, and soil properties. Six rotation treatments were implemented from 2016 to 2023 as follows: rice–wheat (control), rice––rape, rice–hairy vetch, rice–barley, rice–faba bean, and rice–winter fallow. Rice growth parameters, yield components, biomass accumulation, and soil properties were measured. Results showed that legume-based rotations, particularly rice–faba bean and rice–hairy vetch, significantly improved rice growth and yield compared to the rice–wheat control. The rice–faba bean rotation increased yield by 19.1% to 8.73 t/ha compared to 7.33 t/ha for the control, while rice–hairy vetch increased yield by 11.9% to 8.20 t/ha. These rotations also demonstrated higher biomass production efficiency, with increases of 33.33% and 25.00%, respectively, in spring crop biomass. Soil nutrients improvements were observed, particularly in available nitrogen, potassium, and electrical conductivity. Legume-based rotations increased the available nitrogen by up to 35.9% compared to the control. The study highlights the potential of diversified crop rotations, especially those incorporating legumes, to enhance rice productivity and soil health in subtropical regions. These findings have important implications for developing sustainable and resilient rice-based cropping systems to address challenges of food security and environmental sustainability in the face of climate change and resource constraints.
Full article
(This article belongs to the Special Issue Effects of Conservation Tillage on Crop Cultivation and Production)
Open AccessArticle
Volatile Distribution in Flowers of Lathyrus odoratus L. by HS-SPME-GC Technique and Enantiomeric Separation Data
by
James Calva, Mayerly Parra and Ángel Benítez
Plants 2024, 13(23), 3272; https://doi.org/10.3390/plants13233272 - 21 Nov 2024
Abstract
Lathyrus odoratus L., commonly known as sweet pea, is a plant with a distinctive aroma that can develop in various habitats. An analysis of the aromatic profile of the species was conducted using the HS-SPME (solid-phase microextraction headspace) technique. This study aimed to
[...] Read more.
Lathyrus odoratus L., commonly known as sweet pea, is a plant with a distinctive aroma that can develop in various habitats. An analysis of the aromatic profile of the species was conducted using the HS-SPME (solid-phase microextraction headspace) technique. This study aimed to explore the composition of and variation in the floral scent emissions of L. odorathus. The floral scents from fresh flowers were collected over different months and analyzed using gas chromatography coupled with mass spectrometry on apolar and polar stationary phase columns. In the apolar column, the majority compounds included linalool (19.27–5.79%), α-trans-bergamotene (29.4–14.21%), and phenyl ethyl alcohol (30.01–1.56%), while on the polar column, the predominant compounds included myrcene (13.25%), (E,E)-α-farnesene (26.33–8.16%), α-trans-bergamotene (42.09–24.82%), and others. This investigation was complemented by enantioselective analysis using a chiral phase based in cyclodextrins, which revealed the presence of (1R)-(+)-α-pinene, (S)-(−)-limonene, (R)-(+)-germacrene D, and (R)-(E)-nerolidol as enantiomerically pure components and linalool as a racemic mixture. Notably, the principal component analysis (PCA) and heatmap revealed variations among the chemical compounds collected at different harvest times. This demonstrates that temporal factors indeed impact chemical compound production. Furthermore, research on the aromatic properties of flowers provides a theoretical basis for studying and improving the components of their scent.
Full article
(This article belongs to the Special Issue Phytochemical Analyses of Secondary Metabolites of Aromatic, Medicinal and Food Plants)
Open AccessReview
The Applications of Plant Polyphenols: Implications for the Development and Biotechnological Utilization of Ilex Species
by
Gong Cheng, Yuxiao Yan, Bingsong Zheng and Daoliang Yan
Plants 2024, 13(23), 3271; https://doi.org/10.3390/plants13233271 - 21 Nov 2024
Abstract
Plants belonging to the Ilex species are distinguished by their rich composition of diverse phenolic compounds and various bioactive substances, which demonstrate dual functionalities in therapeutic applications and health promotion. In recent years, these plants have garnered significant interest among researchers. While the
[...] Read more.
Plants belonging to the Ilex species are distinguished by their rich composition of diverse phenolic compounds and various bioactive substances, which demonstrate dual functionalities in therapeutic applications and health promotion. In recent years, these plants have garnered significant interest among researchers. While the application scope of plant polyphenols (PPs) is extensive, the exploration and utilization of holly polyphenols (HPs) remain comparatively underexplored. This article reviews the research advancements regarding the predominant phenolic compounds present in commonly studied Ilex species over the past five years and summarizes the application studies of PPs across various domains, including pharmacological applications, food technology, health supplements, and cosmetic formulations. The objective of this review is to provide insights into the systematic research and development of HPs, offering references and recommendations to enhance their value.
Full article
(This article belongs to the Special Issue Biological Activities of Plant Extracts 2nd Edition)
►▼
Show Figures
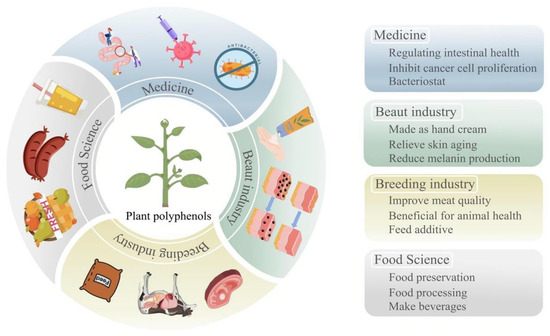
Figure 1
Figure 1
<p>Current status of PP development and utilization. The blue section indicates applications in the field of medicine. The green section indicates applications in the field of beauty industry. The yellow section indicates applications in the field of breeding industry. The gray section indicates applications in the field of food science.</p> Full article ">Figure 2
<p>Methods to enhance the quality, yield, and utilization rate of HPs. The blue section indicates the use of plant growth regulators. The yellow section represents improvements in extraction techniques. The gray section denotes alterations in light conditions. The orange section reflects enhancements in soil quality. The green section signifies the application of solution treatments. The brownish-yellow section indicates the utilization of microencapsulation technology.</p> Full article ">
<p>Current status of PP development and utilization. The blue section indicates applications in the field of medicine. The green section indicates applications in the field of beauty industry. The yellow section indicates applications in the field of breeding industry. The gray section indicates applications in the field of food science.</p> Full article ">Figure 2
<p>Methods to enhance the quality, yield, and utilization rate of HPs. The blue section indicates the use of plant growth regulators. The yellow section represents improvements in extraction techniques. The gray section denotes alterations in light conditions. The orange section reflects enhancements in soil quality. The green section signifies the application of solution treatments. The brownish-yellow section indicates the utilization of microencapsulation technology.</p> Full article ">
Open AccessArticle
Smart Automatic Irrigation Enhances Sap Flow, Growth, and Water Use Efficiency in Containerized Prunus × yedoensis Matsum. Seedling
by
Eon-Ju Jin, Myung-Suk Choi, Hyeok Lee, Eun-Ji Bae, Do-Hyun Kim and Jun-Hyuck Yoon
Plants 2024, 13(23), 3270; https://doi.org/10.3390/plants13233270 - 21 Nov 2024
Abstract
This study conducted a comparative analysis on the effects of smart automatic and semi-automatic irrigation methods on the physiological characteristics and growth of Prunus × yedoensis Matsum. seedlings. The smart automatic irrigation system, which activates irrigation when the soil moisture drops below 15%,
[...] Read more.
This study conducted a comparative analysis on the effects of smart automatic and semi-automatic irrigation methods on the physiological characteristics and growth of Prunus × yedoensis Matsum. seedlings. The smart automatic irrigation system, which activates irrigation when the soil moisture drops below 15%, demonstrated superior characteristics in sap-wood area and bark ratio, as well as excellent water management efficiency, compared to the semi-automatic irrigation method, which involves watering (2.0 L) for 10 min at 60 min intervals starting at 8 AM every day. The analysis of soil moisture content changes under varying weather conditions and irrigation methods showed that smart automatic irrigation effectively maintained optimal moisture levels. Moreover, sap flow in the smart automatic irrigation treatment was more efficiently regulated in response to seasonal variations, showing a strong correlation with climatic factors such as temperature and solar radiation. In contrast, the semi-automatic irrigation treatment led to excessive sap flow during the summer due to a fixed watering schedule, resulting in unnecessary water supply. Analysis of photosynthesis parameters and chlorophyll fluorescence also revealed that smart automatic irrigation achieved higher values in light compensation and saturation points, maximizing photosynthetic efficiency. These findings suggest that the smart automatic irrigation system can enhance plant growth and water use efficiency, contributing to sustainable water management strategies. This research provides critical foundational data for developing efficient agricultural and horticultural irrigation management strategies in response to future climate change.
Full article
(This article belongs to the Section Plant Modeling)
Open AccessArticle
Unconventional Germination in Terrestrial Plants: A Counterintuitive Case in Desiccation-Sensitive Garcinia aristata (Clusiaceae) Seeds Showing Seedling Growth Without Roots
by
Ganesh K. Jaganathan, Jorge A. Sánchez, Mayté Pernús and Baolin Liu
Plants 2024, 13(23), 3269; https://doi.org/10.3390/plants13233269 - 21 Nov 2024
Abstract
Unconventional germination, wherein shoots emerge and establish true leaves before the root emerges, is only found in Zosteraceae. In Garcinia, germination proceeds with the primary root emerging, followed by shoot emergence on the opposite side, but before leaf differentiation, adventitious roots emerge
[...] Read more.
Unconventional germination, wherein shoots emerge and establish true leaves before the root emerges, is only found in Zosteraceae. In Garcinia, germination proceeds with the primary root emerging, followed by shoot emergence on the opposite side, but before leaf differentiation, adventitious roots emerge at the base of the shoots. However, germination and survival mechanisms in several Garcinia species are still unclear. We subjected freshly collected G. aristata seeds to an imbibition test, and germination was evaluated at various temperatures and light conditions. Desiccation sensitivity assessments were made at different stages of drying. The effect of natural drying (ambient storage) on germination was assessed by leaving the seeds outside in natural conditions. Seeds of G. aristata with a moisture content (MC) of 67% had more than 95% germination only at 25 and 25/30 °C both in light/dark and darkness, but at 25/40 °C only 10% germinated. In 4% of the seeds, germination incepted with primary shoot emergence, and a secondary (adventitious) root emerged just before leaf differentiation. More than 95% of the seeds germinated with only a secondary root and shoot emerging concurrently. Drying fresh seeds above silica gel to 30% MC resulted in complete viability loss. Seeds stored at ambient conditions germinated without external water, and had no primary or secondary root, and the emerging shoot continued to grow into seedlings. A root develops in these seeds only when water becomes available. G. aristata seeds are desiccation-sensitive and non-dormant. When no external water is available, G. aristata seeds can germinate with shoots and establish seedlings. This is the first report on germination and successful seedling establishment without roots in Garcinia.
Full article
(This article belongs to the Special Issue Environmental Factors/Compounds and Hormones in Regulation of Seed Dormancy and Germination)
►▼
Show Figures
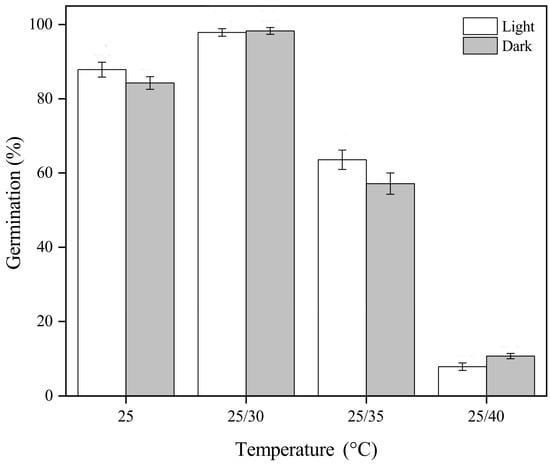
Figure 1
Figure 1
<p>Final germination percentages (mean ± SE of <span class="html-italic">Garcinia aristata</span> seeds incubated under different temperatures and light conditions. Temperature significantly affected germination, while light exposure had no statistically significant impact.</p> Full article ">Figure 2
<p>Cumulative germination percentages (mean ± SE of fresh <span class="html-italic">Garcinia aristata</span> seeds incubated at different temperatures. Different letters indicate significant differences after 30 days when germinated at four different temperatures (<span class="html-italic">p</span> ≤ 0.05). The germination percentage of seeds incubated at 25/40 °C after 30 days (shown with a dotted line) was determined after moving the seeds to 25/30 °C.</p> Full article ">Figure 3
<p>The relationship between moisture content, germination percentage (blue line with error bars), and time taken for first germination (just the error bars) after drying fresh <span class="html-italic">Garcinia aristata</span> seeds for different durations above silica gel. Different lower-case blue letters indicate significant differences between groups for germination. Different lower-case red letters indicate significant differences between groups for mean germination time. Error bars represent standard errors.</p> Full article ">Figure 4
<p>The mean temperature and relative humidity experienced during ‘ambient storage’ of <span class="html-italic">Garcinia aristata</span> seeds. The bars indicate the weekly cumulative germination percentage.</p> Full article ">Figure 5
<p>Two different forms of germination observed in fresh <span class="html-italic">Garcinia aristata</span> seeds with and without primary root. Germination of <span class="html-italic">Garcinia aristata</span> seeds without primary roots (<b>a</b>) 2 days, (<b>b</b>) 7 days, (<b>c</b>) 15 days, and (<b>d</b>) 25 days after germination. Germination of <span class="html-italic">Garcinia aristata</span> seeds with primary and secondary roots: (<b>e</b>) 2 days, (<b>f</b>) 7 days, (<b>g</b>) 15 days, (<b>h</b>) 21 days, and (<b>i</b>) 28 days after germination. S, shoot; AR, adventitious or secondary root; PR, primary root. Scale = 0.5 cm.</p> Full article ">Figure 6
<p>Germination and seedling growth of ‘ambient-stored’ <span class="html-italic">Garcinia aristata</span> seeds with shoots emerging without roots after (<b>a</b>) 14 days, (<b>b</b>) 45 days, and (<b>c</b>) 90 days. The seedlings established fully functional leaves without roots. S, shoot; L, leaves. Scale = 0.5 cm.</p> Full article ">
<p>Final germination percentages (mean ± SE of <span class="html-italic">Garcinia aristata</span> seeds incubated under different temperatures and light conditions. Temperature significantly affected germination, while light exposure had no statistically significant impact.</p> Full article ">Figure 2
<p>Cumulative germination percentages (mean ± SE of fresh <span class="html-italic">Garcinia aristata</span> seeds incubated at different temperatures. Different letters indicate significant differences after 30 days when germinated at four different temperatures (<span class="html-italic">p</span> ≤ 0.05). The germination percentage of seeds incubated at 25/40 °C after 30 days (shown with a dotted line) was determined after moving the seeds to 25/30 °C.</p> Full article ">Figure 3
<p>The relationship between moisture content, germination percentage (blue line with error bars), and time taken for first germination (just the error bars) after drying fresh <span class="html-italic">Garcinia aristata</span> seeds for different durations above silica gel. Different lower-case blue letters indicate significant differences between groups for germination. Different lower-case red letters indicate significant differences between groups for mean germination time. Error bars represent standard errors.</p> Full article ">Figure 4
<p>The mean temperature and relative humidity experienced during ‘ambient storage’ of <span class="html-italic">Garcinia aristata</span> seeds. The bars indicate the weekly cumulative germination percentage.</p> Full article ">Figure 5
<p>Two different forms of germination observed in fresh <span class="html-italic">Garcinia aristata</span> seeds with and without primary root. Germination of <span class="html-italic">Garcinia aristata</span> seeds without primary roots (<b>a</b>) 2 days, (<b>b</b>) 7 days, (<b>c</b>) 15 days, and (<b>d</b>) 25 days after germination. Germination of <span class="html-italic">Garcinia aristata</span> seeds with primary and secondary roots: (<b>e</b>) 2 days, (<b>f</b>) 7 days, (<b>g</b>) 15 days, (<b>h</b>) 21 days, and (<b>i</b>) 28 days after germination. S, shoot; AR, adventitious or secondary root; PR, primary root. Scale = 0.5 cm.</p> Full article ">Figure 6
<p>Germination and seedling growth of ‘ambient-stored’ <span class="html-italic">Garcinia aristata</span> seeds with shoots emerging without roots after (<b>a</b>) 14 days, (<b>b</b>) 45 days, and (<b>c</b>) 90 days. The seedlings established fully functional leaves without roots. S, shoot; L, leaves. Scale = 0.5 cm.</p> Full article ">
Open AccessReview
Roles of Phyllosphere Microbes in Rice Health and Productivity
by
Andrews Danso Ofori, Wei Su, Tengda Zheng, Osmond Datsomor, John Kwame Titriku, Xing Xiang, Abdul Ghani Kandhro, Muhammad Irfan Ahmed, Edzesi Wisdom Mawuli, Richard Tuyee Awuah and Aiping Zheng
Plants 2024, 13(23), 3268; https://doi.org/10.3390/plants13233268 - 21 Nov 2024
Abstract
The phyllosphere, comprising the aerial portions of plants, is a vibrant ecosystem teeming with diverse microorganisms crucial for plant health and productivity. This review examines the functional roles of phyllosphere microorganisms in rice (Oryza sativa), focusing on their importance in nutrient
[...] Read more.
The phyllosphere, comprising the aerial portions of plants, is a vibrant ecosystem teeming with diverse microorganisms crucial for plant health and productivity. This review examines the functional roles of phyllosphere microorganisms in rice (Oryza sativa), focusing on their importance in nutrient uptake, disease resistance, and growth promotion. The molecular mechanisms underlying these interactions are explored along with their potential applications in enhancing sustainable rice production. The symbiotic relationships between rice plants and their associated microorganisms are highlighted, offering insights into improved agricultural practices. Furthermore, this review addresses the challenges and future developments in translating laboratory findings into practical applications. By synthesizing current research, this comprehensive analysis serves as a valuable resource for leveraging phyllosphere microbes in rice farming and related fields.
Full article
(This article belongs to the Section Plant Protection and Biotic Interactions)
Open AccessArticle
Effects of pH, Temperature, and Light on the Inorganic Carbon Uptake Strategies in Early Life Stages of Macrocystis pyrifera (Ochrophyta, Laminariales)
by
Bárbara S. Labbé, Pamela A. Fernández, July Z. Florez and Alejandro H. Buschmann
Plants 2024, 13(23), 3267; https://doi.org/10.3390/plants13233267 - 21 Nov 2024
Abstract
The responses of seaweed species to increased CO2 and lowered pH (Ocean Acidification: OA) depend on their carbon concentrating mechanisms (CCMs) and inorganic carbon (Ci) preferences. However, few studies have described these mechanisms in the early life stages of seaweeds or assessed
[...] Read more.
The responses of seaweed species to increased CO2 and lowered pH (Ocean Acidification: OA) depend on their carbon concentrating mechanisms (CCMs) and inorganic carbon (Ci) preferences. However, few studies have described these mechanisms in the early life stages of seaweeds or assessed the effects of OA and its interactions with other environmental drivers on their functionality and photophysiology. Our study evaluated the effects of pH, light (PAR), temperature, and their interactions on the Ci uptake strategies and photophysiology in the early stages of Macrocystis pyrifera. Gametophytes were cultivated under varying pH (7.80 and 8.20), light (20 and 50 µmol photons m−2s−1), and temperature (12 and 16 °C) conditions for 25 days. We assessed photophysiological responses and CCMs (in particular, the extracellular dehydration of HCO3− to CO2 mediated by the enzyme carbonic anhydrase (CA) and direct HCO3− uptake via an anion exchange port). This study is the first to describe the Ci uptake strategies in gametophytes of M. pyrifera, demonstrating that their primary CCM is the extracellular conversion of HCO3− to CO2 mediated by CA. Additionally, our results indicate that decreased pH can positively affect their photosynthetic efficiency and maximum quantum yield; however, this response is dependent on the light and temperature conditions.
Full article
(This article belongs to the Special Issue Advances in Algal Photosynthesis and Phytochemistry)
►▼
Show Figures
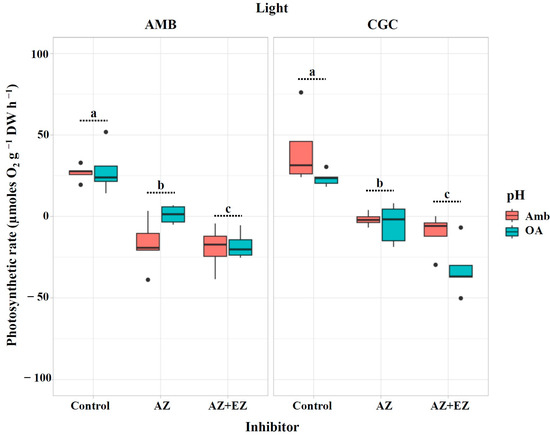
Figure 1
Figure 1
<p>Photosynthetic rate (µmoles O<sub>2</sub> g<sup>−1</sup> DW h<sup>−1</sup>) in <span class="html-italic">M. pyrifera</span> gametophytes after injection of CA inhibitors from Experiment 1: pH × light. Color of legends indicates pH conditions: Ambient (Amb, red) and Ocean Acidification (OA, blue). Light condition panels: AMB Light (50 µmol photon m<sup>−2</sup>s<sup>−1</sup>) and CGC Light (20 µmol photon m<sup>−2</sup>s<sup>−1</sup>). Control: gametophytes without the inhibitors AZ or EZ. Values represent the mean ± SD (<span class="html-italic">p</span> < 0.005, three-way ANOVA). Different letters indicate significantly different values (<span class="html-italic">p</span> < 0.05).</p> Full article ">Figure 2
<p>Photosynthetic rate (µmoles O<sub>2</sub> g<sup>−1</sup> DW h<sup>−1</sup>) in <span class="html-italic">M. pyrifera</span> gametophytes after injection of the direct HCO<sub>3</sub><sup>−</sup> uptake inhibitor from Experiment 1: pH × light. Color of legends indicates pH conditions: Ambient (Amb, red) and Ocean Acidification (OA, blue). Light condition panels: AMB Light (50 µmol photon m<sup>−2</sup>s<sup>−1</sup>) and CGC Light (20 µmol photon m<sup>−2</sup>s<sup>−1</sup>). Control: gametophytes without the inhibitor DIDS. Values represent the mean ± SD (<span class="html-italic">p</span> < 0.005, three-way ANOVA). Different letters indicate significantly different values (<span class="html-italic">p</span> < 0.05).</p> Full article ">Figure 3
<p>Maximum quantum yield (F<sub>v</sub>/F<sub>m</sub>) in <span class="html-italic">M. pyrifera</span> gametophytes in Experiment 1 (corresponding to pH × light). pH conditions: Ambient (AMB) and Ocean Acidification (OA). Color of legends indicates temperature conditions: Ambient (Amb, 12 °C) and Climatic Global Changes (CGC, 16 °C). Values represent the mean ± SD (<span class="html-italic">p</span> < 0.005, two-way ANOVA). Different letters indicate significantly different values (<span class="html-italic">p</span> < 0.05).</p> Full article ">Figure 4
<p>Photosynthetic rate (µmoles O<sub>2</sub> g<sup>−1</sup> DW h<sup>−1</sup>) in <span class="html-italic">M. pyrifera</span> gametophytes after injection of CA inhibitors in Experiment 2: pH × temperature. Color of legends indicates pH conditions: Ambient (Amb, red) and Ocean Acidification (OA, blue). Temperature condition panels: AMB (12 °C) and CGC (16 °C). Control: gametophytes without inhibitors (AZ and EZ). Values represent the mean ± SD (<span class="html-italic">p</span> < 0.005, three-way ANOVA). Different letters indicate significantly different values (<span class="html-italic">p</span> < 0.05).</p> Full article ">Figure 5
<p>Photosynthetic rate (µmoles O<sub>2</sub> g<sup>−1</sup> DW h<sup>−1</sup>) in <span class="html-italic">M. pyrifera</span> gametophytes after injection of the direct HCO<sub>3</sub><sup>−</sup> uptake inhibitor in Experiment 2: pH × temperature. Color of legends indicates pH conditions: Ambient (Amb, red) and Ocean Acidification (OA, blue). Temperature condition panels: AMB (12 °C) and CGC (16 °C). Control: gametophytes without inhibitor (DIDS). Values represent the mean ± SD (<span class="html-italic">p</span> < 0.005, three-way ANOVA). Different letters indicate significantly different values (<span class="html-italic">p</span> < 0.05).</p> Full article ">Figure 6
<p>Maximum quantum yield (F<sub>v</sub>/F<sub>m</sub>) in <span class="html-italic">M. pyrifera</span> gametophytes from Experiment 2 corresponding to pH × temperature. pH conditions: Ambient (AMB) and Ocean Acidification (OA). Color of legends indicates temperature conditions: Ambient (Amb, 12 °C) and Climatic Global Changes (CGC, 16 °C). Values represent the mean ± SD (<span class="html-italic">p</span> < 0.005, two-way ANOVA). Different letters indicate significantly different values (<span class="html-italic">p</span> < 0.05).</p> Full article ">Figure 7
<p>Sampling site of <span class="html-italic">Macrocystis pyrifera</span> sporophylls. (<b>A</b>) Map of Chile showing the study area. (<b>B</b>) Map of Southern Los Lagos Region with a zoom to Carelmapu locality (box). (<b>C</b>) Map of Carelmapu that indicates the specific study area (red point).</p> Full article ">
<p>Photosynthetic rate (µmoles O<sub>2</sub> g<sup>−1</sup> DW h<sup>−1</sup>) in <span class="html-italic">M. pyrifera</span> gametophytes after injection of CA inhibitors from Experiment 1: pH × light. Color of legends indicates pH conditions: Ambient (Amb, red) and Ocean Acidification (OA, blue). Light condition panels: AMB Light (50 µmol photon m<sup>−2</sup>s<sup>−1</sup>) and CGC Light (20 µmol photon m<sup>−2</sup>s<sup>−1</sup>). Control: gametophytes without the inhibitors AZ or EZ. Values represent the mean ± SD (<span class="html-italic">p</span> < 0.005, three-way ANOVA). Different letters indicate significantly different values (<span class="html-italic">p</span> < 0.05).</p> Full article ">Figure 2
<p>Photosynthetic rate (µmoles O<sub>2</sub> g<sup>−1</sup> DW h<sup>−1</sup>) in <span class="html-italic">M. pyrifera</span> gametophytes after injection of the direct HCO<sub>3</sub><sup>−</sup> uptake inhibitor from Experiment 1: pH × light. Color of legends indicates pH conditions: Ambient (Amb, red) and Ocean Acidification (OA, blue). Light condition panels: AMB Light (50 µmol photon m<sup>−2</sup>s<sup>−1</sup>) and CGC Light (20 µmol photon m<sup>−2</sup>s<sup>−1</sup>). Control: gametophytes without the inhibitor DIDS. Values represent the mean ± SD (<span class="html-italic">p</span> < 0.005, three-way ANOVA). Different letters indicate significantly different values (<span class="html-italic">p</span> < 0.05).</p> Full article ">Figure 3
<p>Maximum quantum yield (F<sub>v</sub>/F<sub>m</sub>) in <span class="html-italic">M. pyrifera</span> gametophytes in Experiment 1 (corresponding to pH × light). pH conditions: Ambient (AMB) and Ocean Acidification (OA). Color of legends indicates temperature conditions: Ambient (Amb, 12 °C) and Climatic Global Changes (CGC, 16 °C). Values represent the mean ± SD (<span class="html-italic">p</span> < 0.005, two-way ANOVA). Different letters indicate significantly different values (<span class="html-italic">p</span> < 0.05).</p> Full article ">Figure 4
<p>Photosynthetic rate (µmoles O<sub>2</sub> g<sup>−1</sup> DW h<sup>−1</sup>) in <span class="html-italic">M. pyrifera</span> gametophytes after injection of CA inhibitors in Experiment 2: pH × temperature. Color of legends indicates pH conditions: Ambient (Amb, red) and Ocean Acidification (OA, blue). Temperature condition panels: AMB (12 °C) and CGC (16 °C). Control: gametophytes without inhibitors (AZ and EZ). Values represent the mean ± SD (<span class="html-italic">p</span> < 0.005, three-way ANOVA). Different letters indicate significantly different values (<span class="html-italic">p</span> < 0.05).</p> Full article ">Figure 5
<p>Photosynthetic rate (µmoles O<sub>2</sub> g<sup>−1</sup> DW h<sup>−1</sup>) in <span class="html-italic">M. pyrifera</span> gametophytes after injection of the direct HCO<sub>3</sub><sup>−</sup> uptake inhibitor in Experiment 2: pH × temperature. Color of legends indicates pH conditions: Ambient (Amb, red) and Ocean Acidification (OA, blue). Temperature condition panels: AMB (12 °C) and CGC (16 °C). Control: gametophytes without inhibitor (DIDS). Values represent the mean ± SD (<span class="html-italic">p</span> < 0.005, three-way ANOVA). Different letters indicate significantly different values (<span class="html-italic">p</span> < 0.05).</p> Full article ">Figure 6
<p>Maximum quantum yield (F<sub>v</sub>/F<sub>m</sub>) in <span class="html-italic">M. pyrifera</span> gametophytes from Experiment 2 corresponding to pH × temperature. pH conditions: Ambient (AMB) and Ocean Acidification (OA). Color of legends indicates temperature conditions: Ambient (Amb, 12 °C) and Climatic Global Changes (CGC, 16 °C). Values represent the mean ± SD (<span class="html-italic">p</span> < 0.005, two-way ANOVA). Different letters indicate significantly different values (<span class="html-italic">p</span> < 0.05).</p> Full article ">Figure 7
<p>Sampling site of <span class="html-italic">Macrocystis pyrifera</span> sporophylls. (<b>A</b>) Map of Chile showing the study area. (<b>B</b>) Map of Southern Los Lagos Region with a zoom to Carelmapu locality (box). (<b>C</b>) Map of Carelmapu that indicates the specific study area (red point).</p> Full article ">
Open AccessArticle
Preharvest Application of Exogenous 2,4-Epibrassinolide and Melatonin Enhances the Maturity and Flue-Cured Quality of Tobacco Leaves
by
Kesu Wei, Jiayi Tang, Lei Yang, Shaopeng Chen, Zhijun Cheng, Yijun Yang, Chen Xu, Shengjiang Wu, Yuhang Zhao, Hongmei Di, Ling Li, Dongyang Sun, Jianwei Li and Bo Sun
Plants 2024, 13(23), 3266; https://doi.org/10.3390/plants13233266 - 21 Nov 2024
Abstract
►▼
Show Figures
Tobacco (Nicotiana tabacum) is a globally cultivated crop, with its quality closely associated with the color and chemical composition of cured tobacco leaves. In this experiment, the effects of spraying exogenous 2, 4-epibrassinolide (EBR) and melatonin (MT) on the development of
[...] Read more.
Tobacco (Nicotiana tabacum) is a globally cultivated crop, with its quality closely associated with the color and chemical composition of cured tobacco leaves. In this experiment, the effects of spraying exogenous 2, 4-epibrassinolide (EBR) and melatonin (MT) on the development of tobacco leaves at maturity stage and the quality after curing were investigated. Both EBR and MT treatments significantly enhanced the appearance quality of tobacco leaves at the stem-drying stage. Following preharvest applications, the sugar-to-alkali ratio and potassium content increased, while the contents of starch, total alkaloids, and proteins decreased. The levels of conventional chemical components were improved, enhancing the overall coordination of the tobacco. Transcriptome analysis revealed that EBR treatment down-regulated the chlorophyll biosynthetic genes hemA, MgPEC, and ChlD, while up-regulating the chlorophyll degradation genes CHL2, SGR, and PAOs. Similarly, MT treatment down-regulated the chlorophyll biosynthetic genes FC2 and MgPEC and up-regulated the degradation genes CHL2 and SGR, thus promoting chlorophyll degradation. Furthermore, in the downstream carotenoid biosynthetic pathway, both EBR and MT treatments regulated abscisic acid-related genes, with NCEDs being up-regulated and CYP707A1s down-regulated, thereby promoting the leaf ripening. Metabolomics analysis indicated that EBR treatment primarily regulated alkaloids, terpenoids, and flavonoids, while MT treatment mainly affected flavonoids. Both treatments also reduced the accumulation of the harmful substance aristolochic acid B. Comprehensive evaluations of appearance quality, physiological parameters, transcriptome, and metabolomics analyses demonstrated that exogenous spraying of EBR and MT treatments improved the maturity and quality of cured tobacco leaves, with EBR treatment exhibiting a greater effect than MT treatment.
Full article
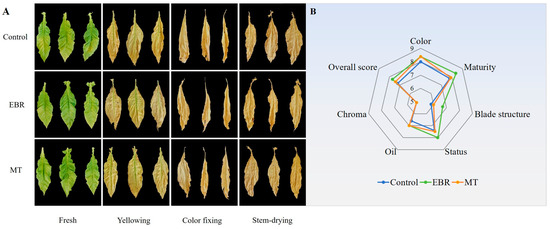
Figure 1
Figure 1
<p>Tobacco during curing stage. (<b>A</b>) The appearance of tobacco during curing stage; (<b>B</b>) The appearance quality evaluation of tobacco leaves at stem-drying stage. EBR, tobacco leaves of EBR-treated; MT, tobacco leaves of MT-treated.</p> Full article ">Figure 2
<p>Pigment content of tobacco leaves during curing stage. (<b>A</b>) Chlorophyll a; (<b>B</b>) Chlorophyll b; (<b>C</b>) Chlorophyll; (<b>D</b>) Carotenoids. EBR, tobacco leaves of EBR-treated; MT, tobacco leaves of MT-treated; F, fresh leaves; Y, yellowing stage; C, color fixing stage; D, stem-drying stage. “a, b, c” in the table mean significant difference among different treatments (<span class="html-italic">p</span> < 0.05).</p> Full article ">Figure 3
<p>Physiological parameters of tobacco leaves during curing stage. (<b>A</b>) Moisture content; (<b>B</b>) Starch; (<b>C</b>) Total sugar; (<b>D</b>) Reducing sugar; (<b>E</b>) Total alkaloid; (<b>F</b>) Sugar-to-alkali ratio; (<b>G</b>) Protein; (<b>H</b>) Chlorinity; (<b>I</b>) Potassium; (<b>J</b>) Chlorogenic acid. EBR, tobacco leaves of EBR-treated; MT, tobacco leaves of MT-treated; F, fresh leaves; Y, yellowing stage; C, color fixing stage; D, stem-drying stage. “a, b” in the table mean significant difference among different treatments (<span class="html-italic">p</span> < 0.05).</p> Full article ">Figure 4
<p>Analysis of gene expression level in transcriptome of fresh tobacco leaves. (<b>A</b>) Co-expression Venn diagram; (<b>B</b>) Number of differential genes; (<b>C</b>) Gene ontology (GO) enrichment analysis of differentially expressed genes (DEGs) in EF/WF; (<b>D</b>) GO enrichment analysis of DEGs in MF/WF; (<b>E</b>) Kyoto encyclopedia of genes and genomes (KEGG) enrichment analysis of DEGs in EF/WF; (<b>F</b>) KEGG enrichment analysis of DEGs in MF/WF. WF, fresh tobacco leaves of control; EF, fresh tobacco leaves of EBR-treated; MF, fresh tobacco leaves of MT-treated.</p> Full article ">Figure 5
<p>The expression of differentially expressed genes (DEGs) in pigment pathway. (<b>A</b>) The expression of DEGs in Porphyrin and chlorophyll metabolism pathway; (<b>B</b>) The expression of DEGs in Carotenoid biosynthesis pathway. EBR, tobacco leaves of EBR-treated; MT, tobacco leaves of MT-treated. “a, b, c” in the table mean significant difference among different treatments (<span class="html-italic">p</span> < 0.05).</p> Full article ">Figure 6
<p>Metabolomic analysis of tobacco leaves at stem-drying stage. (<b>A</b>) Metabolite classification; (<b>B</b>) Kyoto encyclopedia of genes and genomes (KEGG) enrichment analysis of metabolites; (<b>C</b>) Partial least squares discriminant analysis (PLS-DA) plot; (<b>D</b>) Clustering analysis of differentially abundant metabolites (DAMs) in ED/WD; (<b>E</b>) Clustering analysis of DAMs in MD/WD. WD, tobacco leaves of control at stem-drying stage; ED, tobacco leaves of EBR-treated at stem-drying stage; MD, tobacco leaves of MT-treated at stem-drying stage.</p> Full article ">Figure 7
<p>Analysis of co-expressed differentially abundant metabolites (DAMs). (<b>A</b>) Co-expression Venn diagram; (<b>B</b>) Cluster analysis of co-expressed DAMs.</p> Full article ">Figure 8
<p>Correlation analysis between co-expressed differentially abundant metabolites (DAMs) and pigment genes. The octagonal is DAMs, the square is the pigment gene.</p> Full article ">Figure 9
<p>Physiological and molecular regulatory mechanisms by which exogenous 2,4-epibrassinolide and melatonin application enhances tobacco maturity and flue-cured quality. The red arrows indicate increases, while the blue arrows signify decreases in the levels of various substances and gene expression. The green box indicates the effective regulation of EBR treatment, the orange box indicates the effective regulation of MT treatment, and the green and orange mixed boxes indicate that both EBR and MT regulation are effective.</p> Full article ">
<p>Tobacco during curing stage. (<b>A</b>) The appearance of tobacco during curing stage; (<b>B</b>) The appearance quality evaluation of tobacco leaves at stem-drying stage. EBR, tobacco leaves of EBR-treated; MT, tobacco leaves of MT-treated.</p> Full article ">Figure 2
<p>Pigment content of tobacco leaves during curing stage. (<b>A</b>) Chlorophyll a; (<b>B</b>) Chlorophyll b; (<b>C</b>) Chlorophyll; (<b>D</b>) Carotenoids. EBR, tobacco leaves of EBR-treated; MT, tobacco leaves of MT-treated; F, fresh leaves; Y, yellowing stage; C, color fixing stage; D, stem-drying stage. “a, b, c” in the table mean significant difference among different treatments (<span class="html-italic">p</span> < 0.05).</p> Full article ">Figure 3
<p>Physiological parameters of tobacco leaves during curing stage. (<b>A</b>) Moisture content; (<b>B</b>) Starch; (<b>C</b>) Total sugar; (<b>D</b>) Reducing sugar; (<b>E</b>) Total alkaloid; (<b>F</b>) Sugar-to-alkali ratio; (<b>G</b>) Protein; (<b>H</b>) Chlorinity; (<b>I</b>) Potassium; (<b>J</b>) Chlorogenic acid. EBR, tobacco leaves of EBR-treated; MT, tobacco leaves of MT-treated; F, fresh leaves; Y, yellowing stage; C, color fixing stage; D, stem-drying stage. “a, b” in the table mean significant difference among different treatments (<span class="html-italic">p</span> < 0.05).</p> Full article ">Figure 4
<p>Analysis of gene expression level in transcriptome of fresh tobacco leaves. (<b>A</b>) Co-expression Venn diagram; (<b>B</b>) Number of differential genes; (<b>C</b>) Gene ontology (GO) enrichment analysis of differentially expressed genes (DEGs) in EF/WF; (<b>D</b>) GO enrichment analysis of DEGs in MF/WF; (<b>E</b>) Kyoto encyclopedia of genes and genomes (KEGG) enrichment analysis of DEGs in EF/WF; (<b>F</b>) KEGG enrichment analysis of DEGs in MF/WF. WF, fresh tobacco leaves of control; EF, fresh tobacco leaves of EBR-treated; MF, fresh tobacco leaves of MT-treated.</p> Full article ">Figure 5
<p>The expression of differentially expressed genes (DEGs) in pigment pathway. (<b>A</b>) The expression of DEGs in Porphyrin and chlorophyll metabolism pathway; (<b>B</b>) The expression of DEGs in Carotenoid biosynthesis pathway. EBR, tobacco leaves of EBR-treated; MT, tobacco leaves of MT-treated. “a, b, c” in the table mean significant difference among different treatments (<span class="html-italic">p</span> < 0.05).</p> Full article ">Figure 6
<p>Metabolomic analysis of tobacco leaves at stem-drying stage. (<b>A</b>) Metabolite classification; (<b>B</b>) Kyoto encyclopedia of genes and genomes (KEGG) enrichment analysis of metabolites; (<b>C</b>) Partial least squares discriminant analysis (PLS-DA) plot; (<b>D</b>) Clustering analysis of differentially abundant metabolites (DAMs) in ED/WD; (<b>E</b>) Clustering analysis of DAMs in MD/WD. WD, tobacco leaves of control at stem-drying stage; ED, tobacco leaves of EBR-treated at stem-drying stage; MD, tobacco leaves of MT-treated at stem-drying stage.</p> Full article ">Figure 7
<p>Analysis of co-expressed differentially abundant metabolites (DAMs). (<b>A</b>) Co-expression Venn diagram; (<b>B</b>) Cluster analysis of co-expressed DAMs.</p> Full article ">Figure 8
<p>Correlation analysis between co-expressed differentially abundant metabolites (DAMs) and pigment genes. The octagonal is DAMs, the square is the pigment gene.</p> Full article ">Figure 9
<p>Physiological and molecular regulatory mechanisms by which exogenous 2,4-epibrassinolide and melatonin application enhances tobacco maturity and flue-cured quality. The red arrows indicate increases, while the blue arrows signify decreases in the levels of various substances and gene expression. The green box indicates the effective regulation of EBR treatment, the orange box indicates the effective regulation of MT treatment, and the green and orange mixed boxes indicate that both EBR and MT regulation are effective.</p> Full article ">
Open AccessArticle
Phytochemical, Antioxidant, Antimicrobial and Safety Profile of Glycyrrhiza glabra L. Extract Obtained from Romania
by
Iulia Semenescu, Stefana Avram, Diana Similie, Daliana Minda, Zorita Diaconeasa, Delia Muntean, Antonina Evelina Lazar, Daniela Gurgus and Corina Danciu
Plants 2024, 13(23), 3265; https://doi.org/10.3390/plants13233265 - 21 Nov 2024
Abstract
Glycyrrhiza glabra L., also known as licorice, belongs to the Fabaceae family and is one of the most commercially valuable plants worldwide, being used in the pharmaceutical, cosmetic, and food industries, both for its therapeutic benefits as well as for the sweetening properties
[...] Read more.
Glycyrrhiza glabra L., also known as licorice, belongs to the Fabaceae family and is one of the most commercially valuable plants worldwide, being used in the pharmaceutical, cosmetic, and food industries, both for its therapeutic benefits as well as for the sweetening properties of the extract. This study evaluates the phytochemical composition, the biological activities, and the safety profile of a methanolic extract of licorice root (LRE) obtained from Romania. Ten phytocompounds were quantified by the HPLC-DAD-ESI+, the most abundant being the triterpene glycyrrhizin (13.927 mg/g dry extract.), followed by these flavonoids: liquiritin, liquiritigenin-apiosyl-glucoside, and apigenin-rutinoside liquiritigenin. The total phenolic content of the LRE was found to be 169.83 mg gallic acid/g dry extract. (GAE/g d.e.), and the extract showed a maximum of 79.29% antioxidant activity in the 2,2-diphenyl-1-picrylhydrazyl (DPPH) assay. Good antimicrobial activity of the LRE was observed for Gram-negative bacteria, especially for S. pneumoniae and S. pyogenes. The mineral content of the LRE was indicative of the lack of toxicity; heavy metals such as lead, cadmium, arsenic, nickel, and cobalt were below the detection limit. The safety profile of the licorice extract was assessed using the in vivo hen egg test-chorioallantoic membrane (HET-CAM protocol), indicating no irritability, good tolerability, and biocompatibility. The phytochemical and biological characterization of the Romanian licorice root extract reveals a good source of glycyrrhizin and polyphenols with antioxidant and antimicrobial potential, along with a safety profile that may be useful for future therapeutic applications.
Full article
(This article belongs to the Special Issue Insights into Functional and Medicinal Value of Natural Products from Plants)
►▼
Show Figures
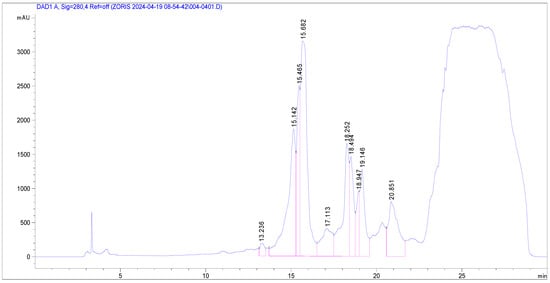
Figure 1
Figure 1
<p>HPLC-PDA chromatograms of <span class="html-italic">G. glabra</span> recorded at 280 nm.</p> Full article ">Figure 2
<p>HPLC-PDA chromatograms of <span class="html-italic">G. glabra</span> recorded at 340 nm.</p> Full article ">Figure 3
<p>DPPH scavenging activity of LRE at various concentrations (µg/mL) and standard antioxidant ascorbic acid (50 µg/mL). Data are expressed as mean ± SD.</p> Full article ">Figure 4
<p>Evaluation of LRE using HET-CAM assay. Stereomicroscopic captures show aspect of chorioallantoic membrane before application (t<sub>0</sub>) and 5 min (t<sub>5</sub>) after application of licorice extract, next to the positive control (SLS), negative control (distilled H<sub>2</sub>O), and solvent control (DMSO); scale bars represent 500 µm.</p> Full article ">
<p>HPLC-PDA chromatograms of <span class="html-italic">G. glabra</span> recorded at 280 nm.</p> Full article ">Figure 2
<p>HPLC-PDA chromatograms of <span class="html-italic">G. glabra</span> recorded at 340 nm.</p> Full article ">Figure 3
<p>DPPH scavenging activity of LRE at various concentrations (µg/mL) and standard antioxidant ascorbic acid (50 µg/mL). Data are expressed as mean ± SD.</p> Full article ">Figure 4
<p>Evaluation of LRE using HET-CAM assay. Stereomicroscopic captures show aspect of chorioallantoic membrane before application (t<sub>0</sub>) and 5 min (t<sub>5</sub>) after application of licorice extract, next to the positive control (SLS), negative control (distilled H<sub>2</sub>O), and solvent control (DMSO); scale bars represent 500 µm.</p> Full article ">
Open AccessArticle
Comprehensive Genome-Wide Analysis of the Receptor-like Protein Gene Family and Functional Analysis of PeRLP8 Associated with Crown Rot Resistance in Passiflora edulis
by
Weijun Yu, Fan Liang, Yue Li, Wenjie Jiang, Yongkang Li, Zitao Shen, Ting Fang and Lihui Zeng
Plants 2024, 13(23), 3264; https://doi.org/10.3390/plants13233264 - 21 Nov 2024
Abstract
Passion fruit (Passiflora edulis Sims) is a Passifloraceae plant with high economic value. Crown rot caused by Rhizoctonia solani is a major fungal disease, which can seriously reduce the yield and quality of passion fruit. Receptor-like proteins (RLPs), which act as pathogen
[...] Read more.
Passion fruit (Passiflora edulis Sims) is a Passifloraceae plant with high economic value. Crown rot caused by Rhizoctonia solani is a major fungal disease, which can seriously reduce the yield and quality of passion fruit. Receptor-like proteins (RLPs), which act as pathogen recognition receptors, are widely involved in plant immune responses and developmental processes. However, the role of RLP family members of passion fruit in resistance to crown rot remains unclear. In this study, evolutionary dynamics analysis and comprehensive genomic characterization of the RLP genes family were performed on passion fruit. A total of 141 PeRLPs in the genome of the ‘Zixiang’ cultivar and 79 PesRLPs in the genome of the ‘Tainong’ cultivar were identified, respectively. Evolutionary analysis showed that proximal and dispersed duplication events were the primary drivers of RLP family expansion. RNA-seq data and RT-qPCR analysis showed that PeRLPs were constitutively expressed in different tissues and induced by low temperature, JA, MeJA, and SA treatments. The PeRLP8 gene was identified as the hub gene by RNA-seq analysis of passion fruit seedlings infected by Rhizoctonia solani. The expression levels of PeRLP8 of the resistant variety Passiflora maliformis (LG) were significantly higher than those of the sensitive variety Passiflora edulis f. flavicarpa (HG). Transient overexpression of PeRLP8 tobacco and passion fruit leaves enhanced the resistance to Rhizoctonia solani, resulting in reduced lesion areas by 52.06% and 54.17%, respectively. In addition, it can increase reactive oxygen species levels and upregulated expression of genes related to active oxygen biosynthesis and JA metabolism in passion fruit leaves. Our research provides new insights into the molecular mechanism and breeding strategy of passion fruit resistance to crown rot.
Full article
(This article belongs to the Special Issue Recent Advances in Horticultural Plant Genomics)
►▼
Show Figures
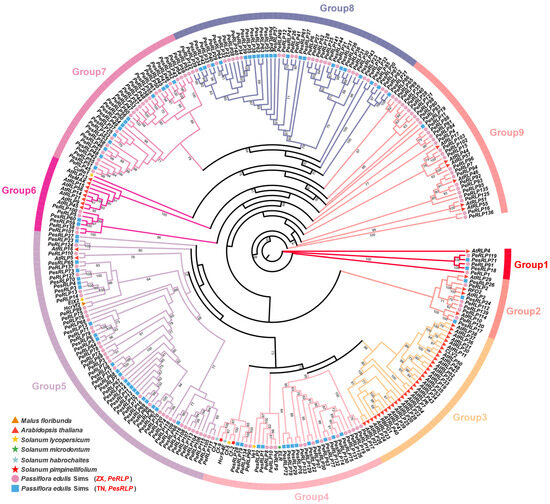
Figure 1
Figure 1
<p>Identification and evolutionary analysis of <span class="html-italic">RLP</span> family genes in passion fruit. Phylogenetic analysis of the RLP homolog proteins from eight plant species, including <span class="html-italic">Malus floribunda</span>, <span class="html-italic">Arabidopsis thaliana</span>, <span class="html-italic">Solanum lycopersicum</span>, <span class="html-italic">Solanum microdontum</span>, <span class="html-italic">Solanum habrochaites</span>, <span class="html-italic">Solanum pimpinellifolium</span>, <span class="html-italic">passiflra edulis</span> Sims (ZX), and <span class="html-italic">passiflra edulis</span> Sims (TN). Each of the eight species was represented by a different shape in the evolutionary tree.</p> Full article ">Figure 2
<p>Gene structure of <span class="html-italic">RLP</span> genes in two passion fruit genomes. The circle represents 141 <span class="html-italic">PeRLP</span> genes in the ZX genome, and the square represents 79 <span class="html-italic">PesRLP</span> genes in the TN genome. The dotted line circles indicated the length of <span class="html-italic">RLP</span> genes.</p> Full article ">Figure 3
<p>Conserved motif analysis of 141 PeRLP and 79 PesRLPs in the ZX and TN genomes. Different color modules indicated different motifs. Different color boxes indicate different conserved domains. The dotted line circles indicated the length of RLP proteins.</p> Full article ">Figure 4
<p>Synteny analysis of <span class="html-italic">RLP</span> genes in four plants, including <span class="html-italic">Arabidopsis</span>, <span class="html-italic">Passiflora edulis</span> Sims (ZX), <span class="html-italic">Passiflora edulis</span> Sims (TN), and <span class="html-italic">Vitis vinifera</span> L. genome. The purple lines display the collinear <span class="html-italic">RLP</span> genes among four plant genomes (<span class="html-italic">Arabidopsis</span>, TN, ZX and <span class="html-italic">Vitis vinifera</span> L.). The light gray lines represented collinear blocks.</p> Full article ">Figure 5
<p>Expression profiles of 141 <span class="html-italic">PeRLP</span> genes. (<b>A</b>) Expression patterns of <span class="html-italic">PeRLP</span> genes in different tissues (root, leaf, seed, and flower) and seedlings of passion fruit with cold treatment. Three biological replicates of HJG (HJGA1, HJGA2, and HJGA3 were recorded as HJGA) and TN (TNA1, TNA2, and TNA3 were recorded as TNA) under normal temperature conditions. Three biological replicates of HJG (HJGB1, HJGB2, and HJGB3 were recorded as HJGB) and TN (TNB1, TNB2, and TNB3 were recorded as TNB) under cold stress. Differences in gene expression changes were shown in color as the scale, mediumvioletred for high expression, and steelblue for low expression. (<b>B</b>,<b>C</b>) Weighted gene co-expression network (WGCNA) was used to identify resistance genes associated with cold-tolerant variety TN. M1-M16 module indicated that the main branches constituted 16 merge modules (based on a threshold of 0.25) and were marked with different colors. Coexpression networks were constructed with eight and one <span class="html-italic">PeRLP</span> hub genes in the M7 and M14 modules, respectively.</p> Full article ">Figure 6
<p>Analysis of gene co-expression network in RNA-seq data of passion fruit infected by <span class="html-italic">R. solani</span>. (<b>A</b>) Transcriptional expression analysis of 141 <span class="html-italic">PeRLP</span> during the infection of resistant variety LG and sensitive variety with <span class="html-italic">R. solani</span>. The heatmap was created based on the log2 (FPKM + 0.01) value of <span class="html-italic">PeRLP</span> genes and normalized by row. Differences in gene expression changes are shown in color as the scale, orange for high expression, and steelblue for low expression. L1, L3, and L5, respectively, represented the 1, 3, and 5 days after the resistant cultivar LG was infected by <span class="html-italic">R. solani</span>. H1, H3, and H5, respectively, represented the 1, 3, and 5 days after the sensitive cultivar HG was infected by <span class="html-italic">R. solani</span>. (<b>B</b>) The correlation analysis of modules and stages by using WGCNA, the heat map showed the correlation between modules and stages. Red and blue indicated positive and negative correlations, respectively. (<b>C</b>) The co-expression gene network was constructed with <span class="html-italic">PeRLP8</span> as the center in the MEblue module. SA signaling, Salicylic acid signaling; ETH biosynthesis and signaling, Ethene biosynthesis and signaling; ABA biosynthesis and catabolism, Abscisic acid biosynthesis and catabolism; JA biosynthesis and signaling, Jasmonic acid biosynthesis and signaling; ROS metabolism, reactive oxygen species metabolism.</p> Full article ">Figure 7
<p>Resistance analysis of <span class="html-italic">PeRLP8</span>-overexpressed passion fruit and tobacco leaves against <span class="html-italic">R. solani.</span> The phenotype observation of transiently overexpressed passion fruit (<b>A</b>) and tobacco (<b>D</b>) leaves after inoculation with <span class="html-italic">R. solani</span>. The dotted lines on the leaves indicate the disease area. Bar = 1 cm. (<b>B</b>,<b>E</b>) The disease area statistics in <span class="html-italic">PeRLP8</span>-overexpressed passion fruit and tobacco leaves. (<b>C</b>,<b>F</b>) The expression levels of <span class="html-italic">PeRLP8</span> in transient-transformed tobacco and passion fruit leaves. (<b>G</b>) ROS content in transient-transformed passion fruit leaves. (<b>H</b>) Quantitative detection of 3 genes co-expressed with <span class="html-italic">PeRLP8</span> in <span class="html-italic">PeRLP8</span>-overexpressed passion fruit leaves. Different letters indicate statistically significant differences compared with the 35S:: GFP control (Student’s <span class="html-italic">t</span>-test, <span class="html-italic">p</span> < 0.05). The error bars represented standard error.</p> Full article ">
<p>Identification and evolutionary analysis of <span class="html-italic">RLP</span> family genes in passion fruit. Phylogenetic analysis of the RLP homolog proteins from eight plant species, including <span class="html-italic">Malus floribunda</span>, <span class="html-italic">Arabidopsis thaliana</span>, <span class="html-italic">Solanum lycopersicum</span>, <span class="html-italic">Solanum microdontum</span>, <span class="html-italic">Solanum habrochaites</span>, <span class="html-italic">Solanum pimpinellifolium</span>, <span class="html-italic">passiflra edulis</span> Sims (ZX), and <span class="html-italic">passiflra edulis</span> Sims (TN). Each of the eight species was represented by a different shape in the evolutionary tree.</p> Full article ">Figure 2
<p>Gene structure of <span class="html-italic">RLP</span> genes in two passion fruit genomes. The circle represents 141 <span class="html-italic">PeRLP</span> genes in the ZX genome, and the square represents 79 <span class="html-italic">PesRLP</span> genes in the TN genome. The dotted line circles indicated the length of <span class="html-italic">RLP</span> genes.</p> Full article ">Figure 3
<p>Conserved motif analysis of 141 PeRLP and 79 PesRLPs in the ZX and TN genomes. Different color modules indicated different motifs. Different color boxes indicate different conserved domains. The dotted line circles indicated the length of RLP proteins.</p> Full article ">Figure 4
<p>Synteny analysis of <span class="html-italic">RLP</span> genes in four plants, including <span class="html-italic">Arabidopsis</span>, <span class="html-italic">Passiflora edulis</span> Sims (ZX), <span class="html-italic">Passiflora edulis</span> Sims (TN), and <span class="html-italic">Vitis vinifera</span> L. genome. The purple lines display the collinear <span class="html-italic">RLP</span> genes among four plant genomes (<span class="html-italic">Arabidopsis</span>, TN, ZX and <span class="html-italic">Vitis vinifera</span> L.). The light gray lines represented collinear blocks.</p> Full article ">Figure 5
<p>Expression profiles of 141 <span class="html-italic">PeRLP</span> genes. (<b>A</b>) Expression patterns of <span class="html-italic">PeRLP</span> genes in different tissues (root, leaf, seed, and flower) and seedlings of passion fruit with cold treatment. Three biological replicates of HJG (HJGA1, HJGA2, and HJGA3 were recorded as HJGA) and TN (TNA1, TNA2, and TNA3 were recorded as TNA) under normal temperature conditions. Three biological replicates of HJG (HJGB1, HJGB2, and HJGB3 were recorded as HJGB) and TN (TNB1, TNB2, and TNB3 were recorded as TNB) under cold stress. Differences in gene expression changes were shown in color as the scale, mediumvioletred for high expression, and steelblue for low expression. (<b>B</b>,<b>C</b>) Weighted gene co-expression network (WGCNA) was used to identify resistance genes associated with cold-tolerant variety TN. M1-M16 module indicated that the main branches constituted 16 merge modules (based on a threshold of 0.25) and were marked with different colors. Coexpression networks were constructed with eight and one <span class="html-italic">PeRLP</span> hub genes in the M7 and M14 modules, respectively.</p> Full article ">Figure 6
<p>Analysis of gene co-expression network in RNA-seq data of passion fruit infected by <span class="html-italic">R. solani</span>. (<b>A</b>) Transcriptional expression analysis of 141 <span class="html-italic">PeRLP</span> during the infection of resistant variety LG and sensitive variety with <span class="html-italic">R. solani</span>. The heatmap was created based on the log2 (FPKM + 0.01) value of <span class="html-italic">PeRLP</span> genes and normalized by row. Differences in gene expression changes are shown in color as the scale, orange for high expression, and steelblue for low expression. L1, L3, and L5, respectively, represented the 1, 3, and 5 days after the resistant cultivar LG was infected by <span class="html-italic">R. solani</span>. H1, H3, and H5, respectively, represented the 1, 3, and 5 days after the sensitive cultivar HG was infected by <span class="html-italic">R. solani</span>. (<b>B</b>) The correlation analysis of modules and stages by using WGCNA, the heat map showed the correlation between modules and stages. Red and blue indicated positive and negative correlations, respectively. (<b>C</b>) The co-expression gene network was constructed with <span class="html-italic">PeRLP8</span> as the center in the MEblue module. SA signaling, Salicylic acid signaling; ETH biosynthesis and signaling, Ethene biosynthesis and signaling; ABA biosynthesis and catabolism, Abscisic acid biosynthesis and catabolism; JA biosynthesis and signaling, Jasmonic acid biosynthesis and signaling; ROS metabolism, reactive oxygen species metabolism.</p> Full article ">Figure 7
<p>Resistance analysis of <span class="html-italic">PeRLP8</span>-overexpressed passion fruit and tobacco leaves against <span class="html-italic">R. solani.</span> The phenotype observation of transiently overexpressed passion fruit (<b>A</b>) and tobacco (<b>D</b>) leaves after inoculation with <span class="html-italic">R. solani</span>. The dotted lines on the leaves indicate the disease area. Bar = 1 cm. (<b>B</b>,<b>E</b>) The disease area statistics in <span class="html-italic">PeRLP8</span>-overexpressed passion fruit and tobacco leaves. (<b>C</b>,<b>F</b>) The expression levels of <span class="html-italic">PeRLP8</span> in transient-transformed tobacco and passion fruit leaves. (<b>G</b>) ROS content in transient-transformed passion fruit leaves. (<b>H</b>) Quantitative detection of 3 genes co-expressed with <span class="html-italic">PeRLP8</span> in <span class="html-italic">PeRLP8</span>-overexpressed passion fruit leaves. Different letters indicate statistically significant differences compared with the 35S:: GFP control (Student’s <span class="html-italic">t</span>-test, <span class="html-italic">p</span> < 0.05). The error bars represented standard error.</p> Full article ">
Open AccessReview
Molecular Basis of Lipid Metabolism in Oryza sativa L.
by
Longxue Chang, Zhichao Liu, Xiaoping Ying, Baxtiyor Kalandarov, Muhammad Ergashev, Xiaohong Tong, Jian Zhang, Jian Jin and Jiezheng Ying
Plants 2024, 13(23), 3263; https://doi.org/10.3390/plants13233263 - 21 Nov 2024
Abstract
Lipids are the basic biological molecules in plants, serving as glycerolipids for cell membranes and triacylglycerols as an energy source. Fatty acids are the major components of plant lipids. Both lipids and fatty acids significantly influence rice quality. Recent studies, through genetic analysis,
[...] Read more.
Lipids are the basic biological molecules in plants, serving as glycerolipids for cell membranes and triacylglycerols as an energy source. Fatty acids are the major components of plant lipids. Both lipids and fatty acids significantly influence rice quality. Recent studies, through genetic analysis, have made significant progress in uncovering the functional mechanisms and regulatory pathways of lipid metabolism including the biological synthesis and degradation of fatty acids, glycerolipids, and triacylglycerols in rice. Meanwhile, quantitative trait loci (QTLs) identified by analyzing the natural variations of the composition and contents of lipids and fatty acids have been integrated and represented on 12 chromosomes. Lipids play multifaceted roles in the growth and development and stress response of rice. Through metabolic engineering and gene-editing technologies, significant advancements have been made in improving the lipid content in rice grains. These studies highlight the understanding the of molecular basis of lipid metabolism and lay a substantial basis for the genetic improvement of rice quality.
Full article
(This article belongs to the Special Issue Research on Plant Genomics and Breeding 2025)
►▼
Show Figures
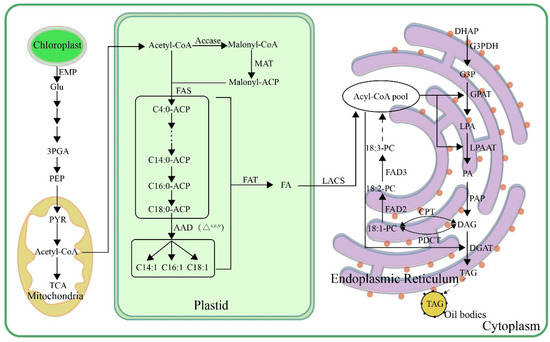
Figure 1
Figure 1
<p>Biosynthetic pathways of TAG in plant seeds. Glu, glucose; 3GPA, 3-phosphoglycerate; PEP, phosphoenolpyruvate; PYR, pyruvic acid; acetyl-CoA, acetyl-coenzyme A; ACCase, acetyl-CoA carboxylase; malonyl-CoA, malonyl-coenzyme A; malonyl-ACP, malonyl-acyl carrier protein; MAT, malonate acyltransferase; FAS, fatty acid synthase; AAD, acyl-ACP desaturase; FAT, acyl-ACP thioesterase; LACS, long-chain acyl-CoA synthetase; DHAP, dihydroxyacetone phosphate; G3PDH, glyceraldehyde-3-phosphate dehydrogenase; G3P, glycerin 3-phosphate; GPAT, glycerol-3-phosphate acyltransferase; LPA, lysophosphatidic acid; LPAAT, lysophosphatidic acid acyltransferase; PA, phosphatidic acid; PAP, phosphatidic acid phosphatase; DAG, diacylglycerol; DGAT, diacylglycerol acyltransferase; TAG, triacylglycerol; PDCT, phosphatidylcholine transferase; CPT, CDP-choline transferase; PC, phosphatidylcholine; FAD2, oleic acid desaturase; FAD3, linoleic acid desaturase.</p> Full article ">Figure 2
<p>Biological metabolic pathways of TAG in plant seeds. LA, lipase; Gly, glycerol; LOX, lipoxygenase; FFA, free fatty acid; ACS, acyl-CoA synthetase; ACD, acyl-CoA dehydrogenase; α,β-ECoA, α,β-enoyl-CoA; ECH, β-enoyl-CoA hydratase; L-β-HoA, L-β-hydroxyacyl-CoA; HCD, L-β-hydroxyacyl-CoA dehydrogenase; β-KCoA, β-ketoacyl-CoA; KCT, β-ketoacyl-CoA thiolase.</p> Full article ">Figure 3
<p>Distribution of QTLs controlling lipid and fatty acid content on 12 chromosomes of rice. Red thick line, centromere.</p> Full article ">
<p>Biosynthetic pathways of TAG in plant seeds. Glu, glucose; 3GPA, 3-phosphoglycerate; PEP, phosphoenolpyruvate; PYR, pyruvic acid; acetyl-CoA, acetyl-coenzyme A; ACCase, acetyl-CoA carboxylase; malonyl-CoA, malonyl-coenzyme A; malonyl-ACP, malonyl-acyl carrier protein; MAT, malonate acyltransferase; FAS, fatty acid synthase; AAD, acyl-ACP desaturase; FAT, acyl-ACP thioesterase; LACS, long-chain acyl-CoA synthetase; DHAP, dihydroxyacetone phosphate; G3PDH, glyceraldehyde-3-phosphate dehydrogenase; G3P, glycerin 3-phosphate; GPAT, glycerol-3-phosphate acyltransferase; LPA, lysophosphatidic acid; LPAAT, lysophosphatidic acid acyltransferase; PA, phosphatidic acid; PAP, phosphatidic acid phosphatase; DAG, diacylglycerol; DGAT, diacylglycerol acyltransferase; TAG, triacylglycerol; PDCT, phosphatidylcholine transferase; CPT, CDP-choline transferase; PC, phosphatidylcholine; FAD2, oleic acid desaturase; FAD3, linoleic acid desaturase.</p> Full article ">Figure 2
<p>Biological metabolic pathways of TAG in plant seeds. LA, lipase; Gly, glycerol; LOX, lipoxygenase; FFA, free fatty acid; ACS, acyl-CoA synthetase; ACD, acyl-CoA dehydrogenase; α,β-ECoA, α,β-enoyl-CoA; ECH, β-enoyl-CoA hydratase; L-β-HoA, L-β-hydroxyacyl-CoA; HCD, L-β-hydroxyacyl-CoA dehydrogenase; β-KCoA, β-ketoacyl-CoA; KCT, β-ketoacyl-CoA thiolase.</p> Full article ">Figure 3
<p>Distribution of QTLs controlling lipid and fatty acid content on 12 chromosomes of rice. Red thick line, centromere.</p> Full article ">
Open AccessArticle
De Novo Transcriptome Assembly of Anoectochilus roxburghii for Morphological Diversity Assessment and Potential Marker Development
by
Wenting Zhang, Ke Chen, Yu Mei and Jihua Wang
Plants 2024, 13(23), 3262; https://doi.org/10.3390/plants13233262 - 21 Nov 2024
Abstract
Anoectochilus roxburghii is a rare and precious medicinal and ornamental plant of Orchidaceae. Abundant morphological characteristics have been observed among cultivated accessions. Our understanding of the genetic basis of morphological diversity is limited due to a lack of sequence data and candidate genes.
[...] Read more.
Anoectochilus roxburghii is a rare and precious medicinal and ornamental plant of Orchidaceae. Abundant morphological characteristics have been observed among cultivated accessions. Our understanding of the genetic basis of morphological diversity is limited due to a lack of sequence data and candidate genes. In this study, a high-quality de novo transcriptome assembly of A.roxburghii was generated. A total of 138,385 unigenes were obtained, and a BUSCO (Benchmarking Universal Single-Copy Orthologs) analysis showed an assembly completeness of 98.8%. Multiple databases were used to obtain a comprehensive annotation, and the unigenes were functionally categorized using the GO (Gene Ontology), KOG (Eukaryotic Orthologous Groups), KEGG (Kyoto Encyclopedia of Genes and Genomes), and Nr databases. After comparing the phenotypic characteristics of five representative cultivars, a set of cultivar-specific, highly expressed unigenes was identified based on a comparative transcriptome analysis. Then, a WGCNA (Weighted Gene Co-expression Network Analysis) was performed to generate gene regulatory modules related to chlorophyll content (red) and sucrose synthase activity (black). In addition, the expression of six and four GO enrichment genes in the red and black modules, respectively, was analyzed using qRT-PCR to determine their putative functional roles in the leaves of the five cultivars. Finally, in silico SSR (Simple Sequence Repeat) mining of the assembled transcriptome identified 44,045 SSRs. Mononucleotide was the most dominant class of SSRs, followed by complex SSRs. In summary, this study reports on the phenomic and genomic resources of A. roxburghii, combining SSR marker development and validation. This report aids in morphological diversity assessments of Anoectochilus roxburghii.
Full article
(This article belongs to the Section Plant Genetics, Genomics and Biotechnology)
►▼
Show Figures
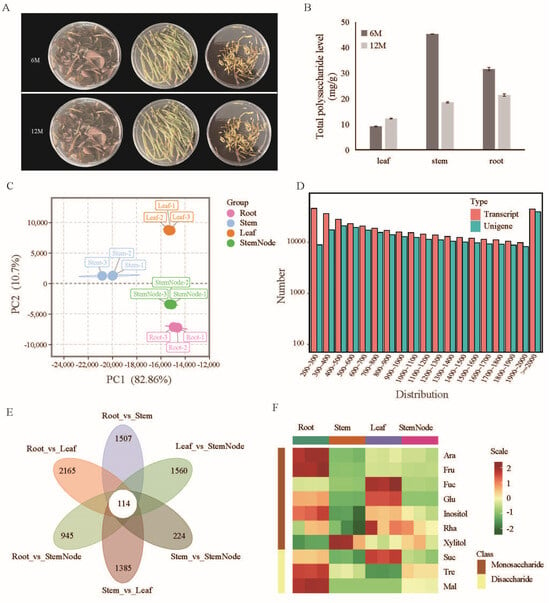
Figure 1
Figure 1
<p>Transcriptome sequencing and sugar composition analysis of <span class="html-italic">A. roxburghii</span> (JXL28). (<b>A</b>) The leaf, stem, and root of JXL28, aged six (up) and twelve (down) months, respectively, are utilized for the assessment of total polysaccharide levels. (<b>B</b>) The total polysaccharide levels in the leaf, stem, and root of <span class="html-italic">A. roxburghii</span> after six and twelve months of growth. (<b>C</b>) A PCA analysis of various tissues of JXL28 transcriptome. (<b>D</b>) Length distribution of assembled transcripts and unigenes of the JXL28 transcriptome. (<b>E</b>) A Venn diagram showing differentially expressed unigenes unique to or shared among differential groups. (<b>F</b>) Heatmap of the levels of ten sugars in various tissues of JXL28. Ara: <span class="html-italic">D</span>-Arabinose; Fru: <span class="html-italic">D</span>-Fructose; Fuc: <span class="html-italic">L</span>-Fucose; Glu: Glucose; Mal: Maltose; Rha: <span class="html-italic">L</span>-Rhamnose; Suc: Sucrose; Tre: Trehalose.</p> Full article ">Figure 2
<p>Functional annotation of unigenes. (<b>A</b>) GO ontology annotation of the <span class="html-italic">A. roxburghii</span> (JXL28) transcriptome showing the major GO terms in the molecular function, biological process, and cellular component categories. (<b>B</b>) Histogram representation of the cluster of orthologous group (COG) classification for assembled unigenes. (<b>C</b>) A KEGG analysis of the JXL28 transcriptome showing the top 20 highly represented KEGG pathways. The <span class="html-italic">X</span>-axis indicates the KEGG (Kyoto Encyclopedia of Genes and Genomes) pathways, and the <span class="html-italic">Y</span>-axis indicates the number of transcripts in each pathway. (<b>D</b>) Species-based distribution of blastx matches for each clustered unitranscript of the JXL28 transcriptome. The species with a match < 1% are grouped in the “Other” category.</p> Full article ">Figure 3
<p>Morphological diversity of <span class="html-italic">A. roxburghii.</span> (<b>A</b>) Morphology of leaf adaxial, leaf abaxial, and seedling of five representative “Jinxianlian” cultivars. (<b>B</b>) An analysis of chlorophyll content (<b>up</b>) and sucrose synthase activity (<b>down</b>); analysis of five representative “Jinxianlian” cultivars. (<b>C</b>) Investigation of ten agronomic characteristics, including weight, height, leaf number, diameter, aerial root number, stem node number, leaf length, and leaf width. The hollow circles represent discrete values. (<b>D</b>) Comparison of leaf surface morphology among five representative “Jinxianlian” cultivars using scanning electron microscopy (SEM). ad: adaxial; ab: abaxial.</p> Full article ">Figure 4
<p>Transcriptome analysis of five representative <span class="html-italic">A. roxburghii</span> cultivars. (<b>A</b>) Results of 3D-PCA of five “Jinxianlian” cultivars based on the expression level of all unigenes, with each dot representing an independent experimental repeat. (<b>B</b>) Numbers of up- and down-regulated DEGs in each comparison (others vs. JXL28). (<b>C</b>) Venn diagram showing the number of DEGs in each combination. (<b>D</b>) Results of five main clusters from <span class="html-italic">K</span>-means clustering analysis. (<b>E</b>) RNA-seq results for several transcription factor candidate from five main clusters.</p> Full article ">Figure 5
<p>Identification of important modules and biomarkers based on a WGCNA. (<b>A</b>) A cluster dendrogram and the color display of co-expression network modules for all unigenes. (<b>B</b>) A correlation matrix of the module eigengene values obtained from the WGCNA. Nine modules were identified, and each module eigengene was tested for correlation with traits. In each cell, the upper values are the correlation coefficients between the module eigengenes and the traits; the lower values are the corresponding <span class="html-italic">p</span>-values; the co-expression modules significantly associated with the content of Chl a, Chl b, and total chlorophyll content and sucrose synthase activity are highlighted in red boxes. (<b>C</b>,<b>D</b>) A scatterplot describing the relationship between MM and GS in the red (<b>C</b>) and black (<b>D</b>) modules; key genes are screened out in the upper-right area, where GS > 0.8 and MM > 0.8. (<b>E</b>,<b>F</b>) A heatmap of the genes in the red (<b>E</b>) and black (<b>F</b>) modules; (<b>G</b>,<b>H</b>) A dotplot of the GO enrichment analysis of the genes in the red (<b>G</b>) and black (<b>H</b>) modules.</p> Full article ">Figure 6
<p>Verification of RNA-seq results via qRT-PCR of candidate unigenes. (<b>A</b>) Six unigenes selected from the hub gene of the red module. (<b>B</b>) Two unigenes selected from the hub gene of the black module. (<b>C</b>) One unigene selected from the DEGs. Error bars indicate SD (n = 3).</p> Full article ">Figure 7
<p>Characterization of potential simple sequence repeat (SSR) markers using MISA software. (<b>A</b>) The distribution of the different nucleotide repeat types (complex; Mono—mononucleotide; Di—dinucleotide; Tr—trinucleotide; Tetra—tetranucleotide; Penta—pentanucleotide; Hexa—hexanucleotide). (<b>B</b>) A stacked bar chart representing the abundance of trinucleotide repeats. (<b>C</b>) PCR amplification of genic-SSR markers in 20 <span class="html-italic">A. roxburghii</span> genotypes.</p> Full article ">
<p>Transcriptome sequencing and sugar composition analysis of <span class="html-italic">A. roxburghii</span> (JXL28). (<b>A</b>) The leaf, stem, and root of JXL28, aged six (up) and twelve (down) months, respectively, are utilized for the assessment of total polysaccharide levels. (<b>B</b>) The total polysaccharide levels in the leaf, stem, and root of <span class="html-italic">A. roxburghii</span> after six and twelve months of growth. (<b>C</b>) A PCA analysis of various tissues of JXL28 transcriptome. (<b>D</b>) Length distribution of assembled transcripts and unigenes of the JXL28 transcriptome. (<b>E</b>) A Venn diagram showing differentially expressed unigenes unique to or shared among differential groups. (<b>F</b>) Heatmap of the levels of ten sugars in various tissues of JXL28. Ara: <span class="html-italic">D</span>-Arabinose; Fru: <span class="html-italic">D</span>-Fructose; Fuc: <span class="html-italic">L</span>-Fucose; Glu: Glucose; Mal: Maltose; Rha: <span class="html-italic">L</span>-Rhamnose; Suc: Sucrose; Tre: Trehalose.</p> Full article ">Figure 2
<p>Functional annotation of unigenes. (<b>A</b>) GO ontology annotation of the <span class="html-italic">A. roxburghii</span> (JXL28) transcriptome showing the major GO terms in the molecular function, biological process, and cellular component categories. (<b>B</b>) Histogram representation of the cluster of orthologous group (COG) classification for assembled unigenes. (<b>C</b>) A KEGG analysis of the JXL28 transcriptome showing the top 20 highly represented KEGG pathways. The <span class="html-italic">X</span>-axis indicates the KEGG (Kyoto Encyclopedia of Genes and Genomes) pathways, and the <span class="html-italic">Y</span>-axis indicates the number of transcripts in each pathway. (<b>D</b>) Species-based distribution of blastx matches for each clustered unitranscript of the JXL28 transcriptome. The species with a match < 1% are grouped in the “Other” category.</p> Full article ">Figure 3
<p>Morphological diversity of <span class="html-italic">A. roxburghii.</span> (<b>A</b>) Morphology of leaf adaxial, leaf abaxial, and seedling of five representative “Jinxianlian” cultivars. (<b>B</b>) An analysis of chlorophyll content (<b>up</b>) and sucrose synthase activity (<b>down</b>); analysis of five representative “Jinxianlian” cultivars. (<b>C</b>) Investigation of ten agronomic characteristics, including weight, height, leaf number, diameter, aerial root number, stem node number, leaf length, and leaf width. The hollow circles represent discrete values. (<b>D</b>) Comparison of leaf surface morphology among five representative “Jinxianlian” cultivars using scanning electron microscopy (SEM). ad: adaxial; ab: abaxial.</p> Full article ">Figure 4
<p>Transcriptome analysis of five representative <span class="html-italic">A. roxburghii</span> cultivars. (<b>A</b>) Results of 3D-PCA of five “Jinxianlian” cultivars based on the expression level of all unigenes, with each dot representing an independent experimental repeat. (<b>B</b>) Numbers of up- and down-regulated DEGs in each comparison (others vs. JXL28). (<b>C</b>) Venn diagram showing the number of DEGs in each combination. (<b>D</b>) Results of five main clusters from <span class="html-italic">K</span>-means clustering analysis. (<b>E</b>) RNA-seq results for several transcription factor candidate from five main clusters.</p> Full article ">Figure 5
<p>Identification of important modules and biomarkers based on a WGCNA. (<b>A</b>) A cluster dendrogram and the color display of co-expression network modules for all unigenes. (<b>B</b>) A correlation matrix of the module eigengene values obtained from the WGCNA. Nine modules were identified, and each module eigengene was tested for correlation with traits. In each cell, the upper values are the correlation coefficients between the module eigengenes and the traits; the lower values are the corresponding <span class="html-italic">p</span>-values; the co-expression modules significantly associated with the content of Chl a, Chl b, and total chlorophyll content and sucrose synthase activity are highlighted in red boxes. (<b>C</b>,<b>D</b>) A scatterplot describing the relationship between MM and GS in the red (<b>C</b>) and black (<b>D</b>) modules; key genes are screened out in the upper-right area, where GS > 0.8 and MM > 0.8. (<b>E</b>,<b>F</b>) A heatmap of the genes in the red (<b>E</b>) and black (<b>F</b>) modules; (<b>G</b>,<b>H</b>) A dotplot of the GO enrichment analysis of the genes in the red (<b>G</b>) and black (<b>H</b>) modules.</p> Full article ">Figure 6
<p>Verification of RNA-seq results via qRT-PCR of candidate unigenes. (<b>A</b>) Six unigenes selected from the hub gene of the red module. (<b>B</b>) Two unigenes selected from the hub gene of the black module. (<b>C</b>) One unigene selected from the DEGs. Error bars indicate SD (n = 3).</p> Full article ">Figure 7
<p>Characterization of potential simple sequence repeat (SSR) markers using MISA software. (<b>A</b>) The distribution of the different nucleotide repeat types (complex; Mono—mononucleotide; Di—dinucleotide; Tr—trinucleotide; Tetra—tetranucleotide; Penta—pentanucleotide; Hexa—hexanucleotide). (<b>B</b>) A stacked bar chart representing the abundance of trinucleotide repeats. (<b>C</b>) PCR amplification of genic-SSR markers in 20 <span class="html-italic">A. roxburghii</span> genotypes.</p> Full article ">
Open AccessArticle
Effects of Drought on the Water Use Strategies of Pure and Mixed Shrubs in the Mu Us Sandy Land
by
Qin Gao, Xiaohong Dang, Zhongju Meng, Yang Liu, Jiale Lou, Yu Yan and Xing Zhang
Plants 2024, 13(23), 3261; https://doi.org/10.3390/plants13233261 - 21 Nov 2024
Abstract
Water resources are crucial factors that limit vegetation recovery, and rational planning of silvicultural patterns is essential for the efficient utilization of water in arid and semi-arid regions. This study examined the water utilization strategies of pure shrubs (pure stands of Artemisia ordosica
[...] Read more.
Water resources are crucial factors that limit vegetation recovery, and rational planning of silvicultural patterns is essential for the efficient utilization of water in arid and semi-arid regions. This study examined the water utilization strategies of pure shrubs (pure stands of Artemisia ordosica and pure stands of Salix psammophila) and mixed shrubs (mixed stands of A. ordosica S. psammophila, and mixed stands of A. ordosica Caragana korshinskii) from the rainy to dry seasons using stable isotope techniques and MixSIAR modeling in the Mu Us Sandy Land in the semi-arid region of China. Mixed shrubs were significantly more effective than pure shrubs in utilizing the primary water sypply from the soil layer. During the rainy season in August, shallow soil water was used to a greater extent, contributing 33.78 ± 2.18%, with no significant difference in the contribution proportion. After a brief drought during the transition period in September, there was a significant increase in the use of the primary water-absorbing soil layer across all vegetation types, with a maximum increase of 39.53%. Conversely, during the dry season in October, after an extended drought, the contribution of the primary water supply layer to vegetation water absorption decreased compared with the transition period, with a maximum increase of only 17.88%. The results of this study revealed that variations in water conditions and vegetation configurations influence the water utilization patterns of the vegetation. This study offers a scientific basis and theoretical support for understanding ecological water use, the rationale behind vegetation establishment, and an assessment of plantation community stability in sandy regions.
Full article
(This article belongs to the Section Plant Ecology)
►▼
Show Figures
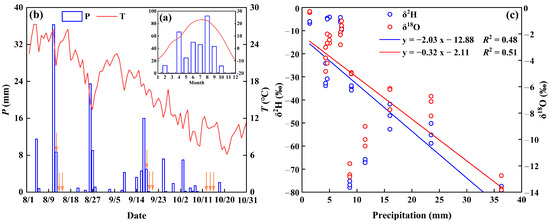
Figure 1
Figure 1
<p>Mean monthly temperature and precipitation for 2023 in the study area (<b>a</b>), temperature and precipitation in the study area during the experimental period (<b>b</b>) (orange arrows indicate when soil vegetation samples were collected), rainfall δ<sup>2</sup>H and δ<sup>18</sup>O variations, and the linear relationship between precipitation and δ<sup>2</sup>H and δ<sup>18</sup>O (<b>c</b>).</p> Full article ">Figure 2
<p>Scatterplots and histograms of δ<sup>2</sup>H and δ<sup>18</sup>Ofor rainwater, soil water, and xylem water in shrub-pure and mixed forests during the measurement period. The boxplot shows the mean (black line), interquartile range (box range), overall range (whisker line), and outliers (black dots). LMWL and GMWL represent the local and global meteoric water lines, respectively. Lowercase letters within the boxes indicate significant differences in δ<sup>2</sup>H and δ<sup>18</sup>O for soil water and xylem water across different silvicultural patterns (<span class="html-italic">p</span> < 0.05).</p> Full article ">Figure 3
<p>Vertical change pattern of soil water content in different plantation forests from the rainy to dry season (0–120 cm) ((<b>a</b>–<b>d</b>) represent <span class="html-italic">Ao, Sp, Ao</span> × <span class="html-italic">Sp,</span> and <span class="html-italic">Ao</span> × <span class="html-italic">Ck</span>, respectively). Capital letters indicate significant differences between the soil depths under the same vegetation cover in the same month (<span class="html-italic">p</span> < 0.05). Lowercase letters indicate significant differences in vegetation cover at the same soil depth during the same month (<span class="html-italic">p</span> < 0.05). Error bars represent standard deviation (mean ± SD, <span class="html-italic">n</span> = 3).</p> Full article ">Figure 4
<p>Distribution patterns of soil water and vegetation xylem water δ<sup>2</sup>H and δ<sup>18</sup>O values in different plantation forests during the experimental period with changes in soil depth and sampling time ((<b>a</b>–<b>d</b>) represent <span class="html-italic">Ao, Sp, Ao × Sp,</span> and <span class="html-italic">Ao</span> × <span class="html-italic">Ck</span>, respectively). Capital letters indicate significant differences between the soil depths under the same vegetation cover in the same month (<span class="html-italic">p</span> < 0.05). Lowercase letters indicate significant differences in vegetation cover at the same soil depth in the same month (<span class="html-italic">p</span> < 0.05). Error bars represent standard deviation (mean ± SD, <span class="html-italic">n</span> = 3).</p> Full article ">Figure 5
<p>Month-to-month variation in water absorption ratio between pure and mixed shrubs in different soil layers based on MixSIAR modeling.</p> Full article ">Figure 6
<p>A thematic map of the study area was prepared based on Landsat 8 imagery. The blue line on the map shows the boundary line of Mu Us Sandy Land, and the red triangle shows the main study area (<b>a</b>). The red dots in the figure show the distribution of sample locations in the study area (<b>b</b>). Examples of four iconic sample plots (<b>c</b>–<b>f</b>).</p> Full article ">Figure 7
<p>Sampling area selection: digging profiles around the base of tree (<b>a</b>). Post-excavation sampling profile (<b>b</b>). 0–10 cm indicates the depth of the soil layer 10 cm down from the soil surface, the rest of the content has this meaning.</p> Full article ">Figure 8
<p>Box plots of stable isotope compositions (δ<sup>2</sup>H and δ<sup>18</sup>O) and lc-excess signature signals in the 0–20, 20–60, and 60–120 cm soil layers of four plantation forests during the study period. The <span class="html-italic">lc-excess</span> was calculated from the local atmospheric precipitation line (LMWL: δ<sup>2</sup>H = 6.39 δ<sup>18</sup>O + 0.56, <span class="html-italic">R</span><sup>2</sup> = 0.94, <span class="html-italic">n</span> = 36). The black spots around the error bar represent outliers. Capital letters on the boxes indicate significant differences between different soil depths under the same vegetation cover for each indicator (<span class="html-italic">p</span> < 0.05).</p> Full article ">Figure 9
<p>Change in the contribution ratio of vegetation water use in the main water-absorbing soil layer from the rainy season to the dry season.</p> Full article ">
<p>Mean monthly temperature and precipitation for 2023 in the study area (<b>a</b>), temperature and precipitation in the study area during the experimental period (<b>b</b>) (orange arrows indicate when soil vegetation samples were collected), rainfall δ<sup>2</sup>H and δ<sup>18</sup>O variations, and the linear relationship between precipitation and δ<sup>2</sup>H and δ<sup>18</sup>O (<b>c</b>).</p> Full article ">Figure 2
<p>Scatterplots and histograms of δ<sup>2</sup>H and δ<sup>18</sup>Ofor rainwater, soil water, and xylem water in shrub-pure and mixed forests during the measurement period. The boxplot shows the mean (black line), interquartile range (box range), overall range (whisker line), and outliers (black dots). LMWL and GMWL represent the local and global meteoric water lines, respectively. Lowercase letters within the boxes indicate significant differences in δ<sup>2</sup>H and δ<sup>18</sup>O for soil water and xylem water across different silvicultural patterns (<span class="html-italic">p</span> < 0.05).</p> Full article ">Figure 3
<p>Vertical change pattern of soil water content in different plantation forests from the rainy to dry season (0–120 cm) ((<b>a</b>–<b>d</b>) represent <span class="html-italic">Ao, Sp, Ao</span> × <span class="html-italic">Sp,</span> and <span class="html-italic">Ao</span> × <span class="html-italic">Ck</span>, respectively). Capital letters indicate significant differences between the soil depths under the same vegetation cover in the same month (<span class="html-italic">p</span> < 0.05). Lowercase letters indicate significant differences in vegetation cover at the same soil depth during the same month (<span class="html-italic">p</span> < 0.05). Error bars represent standard deviation (mean ± SD, <span class="html-italic">n</span> = 3).</p> Full article ">Figure 4
<p>Distribution patterns of soil water and vegetation xylem water δ<sup>2</sup>H and δ<sup>18</sup>O values in different plantation forests during the experimental period with changes in soil depth and sampling time ((<b>a</b>–<b>d</b>) represent <span class="html-italic">Ao, Sp, Ao × Sp,</span> and <span class="html-italic">Ao</span> × <span class="html-italic">Ck</span>, respectively). Capital letters indicate significant differences between the soil depths under the same vegetation cover in the same month (<span class="html-italic">p</span> < 0.05). Lowercase letters indicate significant differences in vegetation cover at the same soil depth in the same month (<span class="html-italic">p</span> < 0.05). Error bars represent standard deviation (mean ± SD, <span class="html-italic">n</span> = 3).</p> Full article ">Figure 5
<p>Month-to-month variation in water absorption ratio between pure and mixed shrubs in different soil layers based on MixSIAR modeling.</p> Full article ">Figure 6
<p>A thematic map of the study area was prepared based on Landsat 8 imagery. The blue line on the map shows the boundary line of Mu Us Sandy Land, and the red triangle shows the main study area (<b>a</b>). The red dots in the figure show the distribution of sample locations in the study area (<b>b</b>). Examples of four iconic sample plots (<b>c</b>–<b>f</b>).</p> Full article ">Figure 7
<p>Sampling area selection: digging profiles around the base of tree (<b>a</b>). Post-excavation sampling profile (<b>b</b>). 0–10 cm indicates the depth of the soil layer 10 cm down from the soil surface, the rest of the content has this meaning.</p> Full article ">Figure 8
<p>Box plots of stable isotope compositions (δ<sup>2</sup>H and δ<sup>18</sup>O) and lc-excess signature signals in the 0–20, 20–60, and 60–120 cm soil layers of four plantation forests during the study period. The <span class="html-italic">lc-excess</span> was calculated from the local atmospheric precipitation line (LMWL: δ<sup>2</sup>H = 6.39 δ<sup>18</sup>O + 0.56, <span class="html-italic">R</span><sup>2</sup> = 0.94, <span class="html-italic">n</span> = 36). The black spots around the error bar represent outliers. Capital letters on the boxes indicate significant differences between different soil depths under the same vegetation cover for each indicator (<span class="html-italic">p</span> < 0.05).</p> Full article ">Figure 9
<p>Change in the contribution ratio of vegetation water use in the main water-absorbing soil layer from the rainy season to the dry season.</p> Full article ">
Open AccessArticle
Effects of Rocky Desertification Stress on Oat (Avena sativa L.) Seed Germination and Seedling Growth in the Karst Areas of Southwest China
by
Haiyan Huang, Yuting Yang, Junqin Li, Yang Gao, Xiangtao Wang, Rui Wang, Zijun Zhou, Puchang Wang and Lili Zhao
Plants 2024, 13(22), 3260; https://doi.org/10.3390/plants13223260 - 20 Nov 2024
Abstract
Oat is an important crop widely distributed in temperate zones and is also commonly planted in the karst areas of southwest China. However, due to severe rocky desertification, the complex soil in this area is characterized by high calcium content, alkaline conditions, and
[...] Read more.
Oat is an important crop widely distributed in temperate zones and is also commonly planted in the karst areas of southwest China. However, due to severe rocky desertification, the complex soil in this area is characterized by high calcium content, alkaline conditions, and drought, which significantly negatively impact the growth of oat seedlings. To study the adaptability of oats to rocky desertification stress at the seedling stage, we investigated the effects of CaCl2 (0–150 mM), the pH (3–9), and drought stress (PEG-6000 solution at 0 to −0.79 MPa) on seed germination and seedling growth. The results showed that (1) calcium stress had dual effects on seed germination within the range of 5–150 mM CaCl2. Low concentrations of CaCl2 (5 mM) promoted the germination potential, germination rate, germination index, and vigor index of oats, as well as the growth and biomass accumulation of radicles in oat seedlings; however, high concentrations of CaCl2 inhibited these germination parameters. (2) Under drought stress, moderate concentrations of a PEG-6000 solution significantly improved the germination potential and germination rate of oat seeds, but the germination index and vigor index decreased with an increasing PEG-6000 concentration. When the PEG-6000 concentration corresponded to −0.06 MPa, the root growth and fresh weight accumulation of oat seedlings were significantly promoted; however, as the concentration increased to −0.53 MPa and –0.79 MPa, seed germination and seedling growth were significantly inhibited. (3) pH treatments had no significant effect on oat seed germination, but all growth indexes of oats showed a downward trend under alkaline conditions. These results suggest that suitable conditions for oat planting in karst rocky desertification areas are 5 mM CaCl2, pH levels of 5–8, and drought stress between 0 and −0.32 MPa. This study provides a theoretical basis for oat introduction, cultivation, and stress-resistant breeding in this area.
Full article
(This article belongs to the Special Issue Ecophysiology and Quality of Crops)
►▼
Show Figures
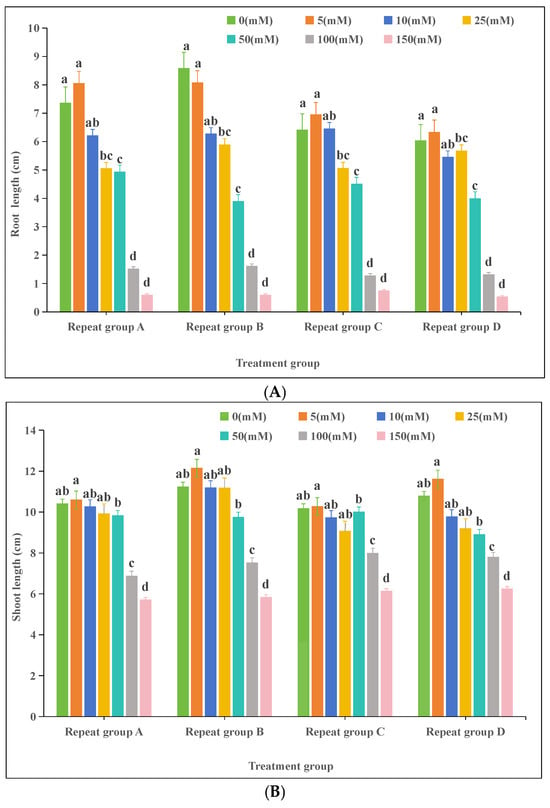
Figure 1
Figure 1
<p>Effects of calcium stress on oat (<span class="html-italic">Avena sativa</span> L.) seedlings: (<b>A</b>) root length; (<b>B</b>) shoot length. Different lowercase letters in the same column indicate significant differences at <span class="html-italic">p</span> < 0.05; the same letter indicates no significant difference (<span class="html-italic">p</span> > 0.05).</p> Full article ">Figure 2
<p>Effects of pH on oat (<span class="html-italic">Avena sativa</span> L.) seedlings: (<b>A</b>) root length; (<b>B</b>) shoot length. Different lowercase letters in the same column indicate significant differences at <span class="html-italic">p</span> < 0.05; the same letter indicates no significant difference (<span class="html-italic">p</span> > 0.05).</p> Full article ">Figure 3
<p>Effects of drought stress on oat (<span class="html-italic">Avena sativa</span> L.) seedlings: (<b>A</b>) root length; (<b>B</b>) shoot length. Different lowercase letters in the same column indicate significant differences at <span class="html-italic">p</span> < 0.05; the same letter indicates no significant difference (<span class="html-italic">p</span> > 0.05). Note: the unit of PEG-6000 (0 to −0.79) is MPa.</p> Full article ">Figure 4
<p>Effects of different stresses on oat (<span class="html-italic">Avena sativa</span> L.) biomass and water content: (<b>A</b>) calcium stress; (<b>B</b>) drought stress; (<b>C</b>) pH. FW: fresh weight; DW: dry weight. Data are presented as the mean ± standard error. Different letters indicate significant differences at <span class="html-italic">p</span> < 0.05.</p> Full article ">Figure 5
<p>Pearson correlation analysis of the effects of calcium stress on oat (<span class="html-italic">Avena sativa</span> L.) seed germination. RL: root length; SL: shoot length; GI: germination index; GP: germination potential; VI: vigor index; GR: germination rate; FW: fresh weight; DW: dry weight; TWC: tissue water content. Note: **** indicates highly significant correlation at <span class="html-italic">p</span> ≤ 0.01; ***, ** indicates significant correlation at <span class="html-italic">p</span> ≤ 0.01, * indicates significant correlation at <span class="html-italic">p</span> ≤ 0.05.</p> Full article ">Figure 6
<p>Pearson correlation analysis of the effects of pH stress on oat (<span class="html-italic">Avena sativa</span> L.) seed germination. RL: root length; SL: shoot length; GI: germination index; GP: germination potential; VI: vigor index; GR: germination rate; FW: fresh weight; DW: dry weight; TWC: tissue water content. Note: **** indicates highly significant correlation at <span class="html-italic">p</span> ≤ 0.01; ***, ** indicates significant correlation at <span class="html-italic">p</span> ≤ 0.01, * indicates significant correlation at <span class="html-italic">p</span> ≤ 0.05.</p> Full article ">Figure 7
<p>Pearson correlation analysis of the effects of drought stress on oat (<span class="html-italic">Avena sativa</span> L.) seed germination. RL: root length; SL: shoot length; GI: germination index; GP: germination potential; VI: vigor index; GR: germination rate; FW: fresh weight; DW: dry weight; TWC: tissue water content. Note: **** indicates highly significant correlation at <span class="html-italic">p</span> ≤ 0.01; ***, ** indicates significant correlation at <span class="html-italic">p</span> ≤ 0.01, * indicates significant correlation at <span class="html-italic">p</span> ≤ 0.05.</p> Full article ">
<p>Effects of calcium stress on oat (<span class="html-italic">Avena sativa</span> L.) seedlings: (<b>A</b>) root length; (<b>B</b>) shoot length. Different lowercase letters in the same column indicate significant differences at <span class="html-italic">p</span> < 0.05; the same letter indicates no significant difference (<span class="html-italic">p</span> > 0.05).</p> Full article ">Figure 2
<p>Effects of pH on oat (<span class="html-italic">Avena sativa</span> L.) seedlings: (<b>A</b>) root length; (<b>B</b>) shoot length. Different lowercase letters in the same column indicate significant differences at <span class="html-italic">p</span> < 0.05; the same letter indicates no significant difference (<span class="html-italic">p</span> > 0.05).</p> Full article ">Figure 3
<p>Effects of drought stress on oat (<span class="html-italic">Avena sativa</span> L.) seedlings: (<b>A</b>) root length; (<b>B</b>) shoot length. Different lowercase letters in the same column indicate significant differences at <span class="html-italic">p</span> < 0.05; the same letter indicates no significant difference (<span class="html-italic">p</span> > 0.05). Note: the unit of PEG-6000 (0 to −0.79) is MPa.</p> Full article ">Figure 4
<p>Effects of different stresses on oat (<span class="html-italic">Avena sativa</span> L.) biomass and water content: (<b>A</b>) calcium stress; (<b>B</b>) drought stress; (<b>C</b>) pH. FW: fresh weight; DW: dry weight. Data are presented as the mean ± standard error. Different letters indicate significant differences at <span class="html-italic">p</span> < 0.05.</p> Full article ">Figure 5
<p>Pearson correlation analysis of the effects of calcium stress on oat (<span class="html-italic">Avena sativa</span> L.) seed germination. RL: root length; SL: shoot length; GI: germination index; GP: germination potential; VI: vigor index; GR: germination rate; FW: fresh weight; DW: dry weight; TWC: tissue water content. Note: **** indicates highly significant correlation at <span class="html-italic">p</span> ≤ 0.01; ***, ** indicates significant correlation at <span class="html-italic">p</span> ≤ 0.01, * indicates significant correlation at <span class="html-italic">p</span> ≤ 0.05.</p> Full article ">Figure 6
<p>Pearson correlation analysis of the effects of pH stress on oat (<span class="html-italic">Avena sativa</span> L.) seed germination. RL: root length; SL: shoot length; GI: germination index; GP: germination potential; VI: vigor index; GR: germination rate; FW: fresh weight; DW: dry weight; TWC: tissue water content. Note: **** indicates highly significant correlation at <span class="html-italic">p</span> ≤ 0.01; ***, ** indicates significant correlation at <span class="html-italic">p</span> ≤ 0.01, * indicates significant correlation at <span class="html-italic">p</span> ≤ 0.05.</p> Full article ">Figure 7
<p>Pearson correlation analysis of the effects of drought stress on oat (<span class="html-italic">Avena sativa</span> L.) seed germination. RL: root length; SL: shoot length; GI: germination index; GP: germination potential; VI: vigor index; GR: germination rate; FW: fresh weight; DW: dry weight; TWC: tissue water content. Note: **** indicates highly significant correlation at <span class="html-italic">p</span> ≤ 0.01; ***, ** indicates significant correlation at <span class="html-italic">p</span> ≤ 0.01, * indicates significant correlation at <span class="html-italic">p</span> ≤ 0.05.</p> Full article ">
Open AccessArticle
Chemical and Biological Properties of Different Romanian Populations of Hyssopus officinalis Correlated via Molecular Docking
by
Ilinca Merima Imbrea, Magdalena Osiceanu, Anca Hulea, Mukhtar Adeiza Suleiman, Iuliana Popescu, Doris Floares (Oarga), Emilian Onisan, Alina-Georgeta Neacșu, Cosmin Alin Popescu, Calin Hulea, Georgeta Pop, Simona Niță, Florin Imbrea and Diana Obistioiu
Plants 2024, 13(22), 3259; https://doi.org/10.3390/plants13223259 - 20 Nov 2024
Abstract
This study compares three Romanian Hyssopus officinalis species—H. officinalis f. ruber (HOR), H. officinalis f. albus (HOA), and H. officinalis f. cyaneus (HOC)—evaluating their chemical composition and biological activities, specifically protein denaturation, haemolysis inhibition, and antibacterial effects. Chemical profiles were determined using
[...] Read more.
This study compares three Romanian Hyssopus officinalis species—H. officinalis f. ruber (HOR), H. officinalis f. albus (HOA), and H. officinalis f. cyaneus (HOC)—evaluating their chemical composition and biological activities, specifically protein denaturation, haemolysis inhibition, and antibacterial effects. Chemical profiles were determined using Gas Chromatography–Mass Spectrometry (GC-MS). The species were cultivated at two distinct locations: the Didactic and Experimental Station DESUSVT and the Agricultural Research and Development Station Lovrin (ARDSL). This study investigates the correlation between chemical composition, biological activities, and local climate data at each site. The results show significant variations in chemical profiles, with species and cultivation location influencing the biological activities. H. officinalis f. albus (HOA) exhibited the strongest antimicrobial activity, particularly against Gram-positive bacteria. The molecular docking analysis highlighted key compounds, such as cyclohexene,4-isopropenyl-1-methoxymethoxymethyl and elemol, with binding solid affinities to microbial and inflammatory proteins. This study provides valuable insights into the chemical and biological properties of Hyssopus officinalis, emphasising its potential in combating microbial infections, protein denaturation, and haemolysis inhibition.
Full article
(This article belongs to the Special Issue Chemical Analysis, Bioactivity, and Application of Essential Oils)
►▼
Show Figures
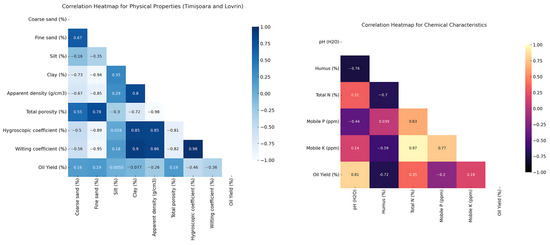
Figure 1
Figure 1
<p>The Pearson correlation matrix for soil physical and chemical characteristics in Timișoara and Lovrin and the influence of oil yield extraction traits.</p> Full article ">Figure 2
<p>The heatmap of Pearson correlation coefficients for chemical composition by variety and location.</p> Full article ">Figure 3
<p>(<b>A</b>) The scree plot of P.C.A.; (<b>B</b>) the biplot of P.C.A.; (<b>C</b>) the contribution of variables to the first dimension of P.C.A.; (<b>D</b>) the contribution of variables to the second dimension of P.C.A. Note: V1: α-Pinene, V2: α-Phellandrene, V3: β-Pinene, V4: Sabinene, V5: β-Myrcene, V6: Limonene, V7: β-Phellandrene, V8: <span class="html-italic">β-cis</span>-Ocimene, V9: Eucalyptol, V10: cyclohexene, 4-isopropenyl-1-methoxymethoxymethyl-, V11: α-Gurjunene, V12: β-Bourbonene, V13: linalool, V14: 3-Thujanone, V15: Caryophyllene, V16: Alloaromadendrene, V17: Humulene, V18: α-Caryophyllene, V19: γ-Cadinene, V20: Pinocamphone, V21: germacrene d, V22: Isocamphopinone, V23: γ-Elemene, V24: Estragole, V25: 3-octen-5-yne, 2,7-dimethyl-, (e)-, V26: p-menth-1-en-8-ol, V27: (1r)-(−)-Myrtenal, V28: myrtenol, V29: Ledol, V30: elemol, V31: Eugenol methyl ether, V32: Caryophyllene oxide, V33: (−)-Spathulenol.</p> Full article ">Figure 4
<p>Three-dimensional (3D) and two-dimensional (2D) structural best binding modes between the antimicrobial, anti-inflammatory proteins and the identified compounds of the Hyssopus plants.</p> Full article ">
<p>The Pearson correlation matrix for soil physical and chemical characteristics in Timișoara and Lovrin and the influence of oil yield extraction traits.</p> Full article ">Figure 2
<p>The heatmap of Pearson correlation coefficients for chemical composition by variety and location.</p> Full article ">Figure 3
<p>(<b>A</b>) The scree plot of P.C.A.; (<b>B</b>) the biplot of P.C.A.; (<b>C</b>) the contribution of variables to the first dimension of P.C.A.; (<b>D</b>) the contribution of variables to the second dimension of P.C.A. Note: V1: α-Pinene, V2: α-Phellandrene, V3: β-Pinene, V4: Sabinene, V5: β-Myrcene, V6: Limonene, V7: β-Phellandrene, V8: <span class="html-italic">β-cis</span>-Ocimene, V9: Eucalyptol, V10: cyclohexene, 4-isopropenyl-1-methoxymethoxymethyl-, V11: α-Gurjunene, V12: β-Bourbonene, V13: linalool, V14: 3-Thujanone, V15: Caryophyllene, V16: Alloaromadendrene, V17: Humulene, V18: α-Caryophyllene, V19: γ-Cadinene, V20: Pinocamphone, V21: germacrene d, V22: Isocamphopinone, V23: γ-Elemene, V24: Estragole, V25: 3-octen-5-yne, 2,7-dimethyl-, (e)-, V26: p-menth-1-en-8-ol, V27: (1r)-(−)-Myrtenal, V28: myrtenol, V29: Ledol, V30: elemol, V31: Eugenol methyl ether, V32: Caryophyllene oxide, V33: (−)-Spathulenol.</p> Full article ">Figure 4
<p>Three-dimensional (3D) and two-dimensional (2D) structural best binding modes between the antimicrobial, anti-inflammatory proteins and the identified compounds of the Hyssopus plants.</p> Full article ">
Open AccessArticle
Development of Analytical Model to Describe Reflectance Spectra in Leaves with Palisade and Spongy Mesophyll
by
Ekaterina Sukhova, Yuriy Zolin, Kseniya Grebneva, Ekaterina Berezina, Oleg Bondarev, Anastasiia Kior, Alyona Popova, Daria Ratnitsyna, Lyubov Yudina and Vladimir Sukhov
Plants 2024, 13(22), 3258; https://doi.org/10.3390/plants13223258 - 20 Nov 2024
Abstract
Remote sensing plays an important role in plant cultivation and ecological monitoring. This sensing is often based on measuring spectra of leaf reflectance, which are dependent on morphological, biochemical, and physiological characteristics of plants. However, interpretation of the reflectance spectra requires the development
[...] Read more.
Remote sensing plays an important role in plant cultivation and ecological monitoring. This sensing is often based on measuring spectra of leaf reflectance, which are dependent on morphological, biochemical, and physiological characteristics of plants. However, interpretation of the reflectance spectra requires the development of new tools to analyze relations between plant characteristics and leaf reflectance. The current study was devoted to the development, parameterization, and verification of the analytical model to describe reflectance spectra of the dicot plant leaf with palisade and spongy mesophyll layers (on the example of pea leaves). Four variables (intensities of forward and backward collimated light and intensities of forward and backward scattered light) were considered. Light reflectance and transmittance on borders of lamina (Snell’s and Fresnel’s laws), light transmittance in the palisade mesophyll (Beer–Bouguer–Lambert law), and light transmittance and scattering in the spongy mesophyll (Kubelka–Munk theory) were described. The developed model was parameterized based on experimental results (reflectance spectra, contents of chlorophylls and carotenoid, and thicknesses of palisade and spongy mesophyll in pea leaves) and the literature data (final R2 was 0.989 for experimental and model-based reflectance spectra). Further model-based and experimental investigations showed that decreasing palisade and spongy mesophyll thicknesses in pea leaves (from 35.5 to 25.2 µm and from 58.6 to 47.8 µm, respectively) increased reflectance of green light and decreased reflectance of near-infrared light. Similarity between model-based and experimental results verified the developed model. Thus, the model can be used to analyze leaf reflectance spectra and, thereby, to increase efficiency of the plant remote and proximal sensing.
Full article
(This article belongs to the Special Issue Integration of Spectroscopic and Photosynthetic Analyses in Plants)
►▼
Show Figures
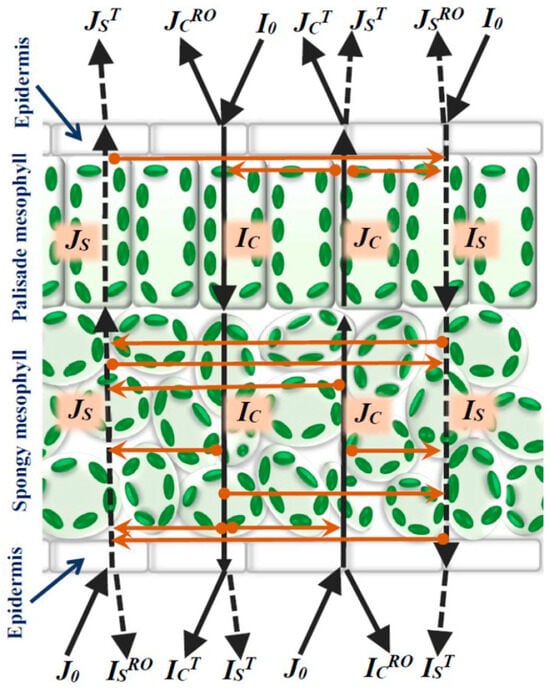
Figure 1
Figure 1
<p>The scheme of light flows in the model of reflectance and transmission in the leaf of a dicot plant. Black continuous lines show forward light flows. Black dotted lines show backward light flows. Orange lines show transformation between light flows. <span class="html-italic">I<sub>C</sub></span> is the forward collimated light, <span class="html-italic">I<sub>S</sub></span> is the forward scattered light, <span class="html-italic">J<sub>C</sub></span> is the backward collimated light, and <span class="html-italic">J<sub>S</sub></span> is the backward scattered light. <span class="html-italic">I<sub>0</sub></span> and <span class="html-italic">J<sub>0</sub></span> are intensities of the forward and backward collimated light directed to adaxial and abaxial leaf surfaces, respectively (the incident light). <span class="html-italic">J<sub>C</sub><sup>RO</sup></span> and <span class="html-italic">I<sub>C</sub><sup>RO</sup></span> are intensities of the collimated light reflecting from adaxial and abaxial leaf surfaces in air. <span class="html-italic">J<sub>S</sub><sup>RO</sup></span> and <span class="html-italic">I<sub>S</sub><sup>RO</sup></span> are intensities of the scattered light reflecting from adaxial and abaxial leaf surfaces in air. <span class="html-italic">J<sub>C</sub><sup>T</sup></span> and <span class="html-italic">I<sub>C</sub><sup>T</sup></span> are intensities of the collimated light transferring from leaf to air across adaxial and abaxial surfaces. <span class="html-italic">J<sub>S</sub><sup>T</sup></span> and <span class="html-italic">I<sub>S</sub><sup>T</sup></span> are intensities of the scattered light transferring from leaf to air across adaxial and abaxial surfaces.</p> Full article ">Figure 2
<p>Image of pea leaf cross-section (<b>a</b>) and average thickness of palisade (<span class="html-italic">h</span>) and spongy (<span class="html-italic">l</span>) mesophyll (<span class="html-italic">n</span> = 6) (<b>b</b>). The second mature leaf was used. Pea plants were cultivated for 16 days under the moderate light intensity.</p> Full article ">Figure 3
<p>Spectra of specific light absorption coefficients for chlorophyll a, chlorophyll b, and carotenoids (on an example of β-carotene) (<b>a</b>) and average concentrations of these pigments in pea leaves (<span class="html-italic">n</span> = 6) (<b>b</b>). The spectra of light absorption were constructed on the basis of [<a href="#B58-plants-13-03258" class="html-bibr">58</a>]. The pigment concentrations were experimentally measured. The second mature leaf was used. Pea plants were cultivated for 16 days under moderate light intensity.</p> Full article ">Figure 4
<p>Spectra of leaf reflectance calculated on basis of the developed model with different quantities of the iterations (<span class="html-italic">N</span>). The leaf reflectance was calculated as a ratio of the sum of all components of backward collimated and backward scattered light transferring through the adaxial leaf surface (<span class="html-italic">J<sub>out</sub></span>) to the intensity of incident light (<span class="html-italic">I</span><sub>0</sub>). The influence of <span class="html-italic">N</span> on <span class="html-italic">J<sub>out</sub></span> was calculated in accordance with Equation (58). The following parameters were used (see <a href="#sec2dot1-plants-13-03258" class="html-sec">Section 2.1</a> for details): <span class="html-italic">β</span><sub>O1</sub> = 35° (in accordance with angle of the leaf illumination by PolyPen RP 410), <span class="html-italic">I<sub>0</sub> </span>= 1000 μmol m<sup>−2</sup>s<sup>−1</sup> and <span class="html-italic">J</span><sub>0</sub> = 0 μmol m<sup>−2</sup>s<sup>−1</sup> (assumed), <span class="html-italic">F<sub>S</sub> </span>= 0 (assumed), <span class="html-italic">n<sub>I</sub> </span>= 1.415 [<a href="#B50-plants-13-03258" class="html-bibr">50</a>], <span class="html-italic">f</span> = 0.5 (assumed), <span class="html-italic">h</span> = 35.5 μm and <span class="html-italic">l</span> = 58.6 μm (<a href="#plants-13-03258-f002" class="html-fig">Figure 2</a>b), <math display="inline"><semantics> <mrow> <msub> <mrow> <mi>s</mi> </mrow> <mrow> <mi>P</mi> </mrow> </msub> <mo>=</mo> </mrow> </semantics></math> 5 cm<sup>−1</sup> and <math display="inline"><semantics> <mrow> <msub> <mrow> <mi>s</mi> </mrow> <mrow> <mi>S</mi> <mi>p</mi> </mrow> </msub> </mrow> </semantics></math> = 1000 cm<sup>−1</sup> [<a href="#B49-plants-13-03258" class="html-bibr">49</a>], C<sub>ChA</sub> = 2.77 mg cm<sup>−3</sup>, C<sub>ChB</sub> = 1.69 mg cm<sup>−3</sup>, and C<sub>Car</sub> = 0.94 mg cm<sup>−3</sup> corresponded to average experimental concentrations of these pigments (1.39, 0.85, and 0.47 mg cm<sup>−3</sup>, respectively, <a href="#plants-13-03258-f003" class="html-fig">Figure 3</a>b) at <span class="html-italic">N<sub>Sp/P</sub> </span>= 0.2 [<a href="#B49-plants-13-03258" class="html-bibr">49</a>]); <math display="inline"><semantics> <mrow> <msub> <mrow> <mi>a</mi> </mrow> <mrow> <mi>C</mi> <mi>h</mi> <mi>A</mi> </mrow> </msub> <mfenced separators="|"> <mrow> <mi>λ</mi> </mrow> </mfenced> </mrow> </semantics></math>, <math display="inline"><semantics> <mrow> <msub> <mrow> <mi>a</mi> </mrow> <mrow> <mi>C</mi> <mi>h</mi> <mi>B</mi> </mrow> </msub> <mfenced separators="|"> <mrow> <mi>λ</mi> </mrow> </mfenced> </mrow> </semantics></math>, and <math display="inline"><semantics> <mrow> <msub> <mrow> <mi>a</mi> </mrow> <mrow> <mi>C</mi> <mi>a</mi> <mi>r</mi> </mrow> </msub> <mfenced separators="|"> <mrow> <mi>λ</mi> </mrow> </mfenced> </mrow> </semantics></math> are shown in <a href="#plants-13-03258-f003" class="html-fig">Figure 3</a>a.</p> Full article ">Figure 5
<p>Experimental and model-based spectra of leaf reflectance. The average experimental reflectance spectrum (<span class="html-italic">n</span> = 6) measured in the second mature leaf of pea plants (PolyPen RP 410) is shown. Standard errors are not shown because they are small. Pea plants were cultivated for 16 days under the moderate light intensity. Parameters of the model are shown in <a href="#plants-13-03258-f004" class="html-fig">Figure 4</a>; <span class="html-italic">N</span> = 6 was used for the analysis. R<sup>2</sup> and RMSE are the determination coefficient and root mean square error between the experimental and model-based spectra.</p> Full article ">Figure 6
<p>Model-based spectra of leaf reflectance at <math display="inline"><semantics> <mrow> <msub> <mrow> <mi>s</mi> </mrow> <mrow> <mi>S</mi> <mi>p</mi> </mrow> </msub> <mo>=</mo> </mrow> </semantics></math> 1000 cm<sup>−1</sup> (<b>a</b>), <math display="inline"><semantics> <mrow> <msub> <mrow> <mi>s</mi> </mrow> <mrow> <mi>S</mi> <mi>p</mi> </mrow> </msub> <mo>=</mo> </mrow> </semantics></math> 800 cm<sup>−1</sup> (<b>b</b>), <math display="inline"><semantics> <mrow> <msub> <mrow> <mi>s</mi> </mrow> <mrow> <mi>S</mi> <mi>p</mi> </mrow> </msub> <mo>=</mo> </mrow> </semantics></math> 600 cm<sup>−1</sup> (<b>c</b>), and <math display="inline"><semantics> <mrow> <msub> <mrow> <mi>s</mi> </mrow> <mrow> <mi>S</mi> <mi>p</mi> </mrow> </msub> <mo>=</mo> </mrow> </semantics></math> 400 cm<sup>−1</sup> (<b>d</b>) and the experimental spectrum (from <a href="#plants-13-03258-f005" class="html-fig">Figure 5</a>). Other parameters of the model were the same as the parameters that were used for the simulation of the spectrum in <a href="#plants-13-03258-f005" class="html-fig">Figure 5</a>. R<sup>2</sup> and RMSE are, respectively, the determination coefficient and root mean square error between the experimental and model-based spectra.</p> Full article ">Figure 7
<p>(<b>a</b>) Model-based spectrum of leaf reflectance at C<sub>ChA</sub> = 3.19 mg cm<sup>−3</sup> and C<sub>ChB</sub> = 2.09 mg cm<sup>−3</sup> and the experimental spectrum (from <a href="#plants-13-03258-f005" class="html-fig">Figure 5</a>). Other parameters of the model were the same as the parameters that were used for the simulation of the spectrum in <a href="#plants-13-03258-f006" class="html-fig">Figure 6</a>c. R<sup>2</sup> and RMSE are, respectively, the determination coefficient and root mean square error between the experimental and model-based spectra. (<b>b</b>) Average concentrations of chlorophyll a and b, which corresponded to C<sub>ChA</sub> = 3.19 mg cm<sup>−3</sup> and C<sub>ChB</sub> = 2.09 mg cm<sup>−3</sup>, and their experimental concentrations in pea leaves (form <a href="#plants-13-03258-f003" class="html-fig">Figure 3</a>).</p> Full article ">Figure 8
<p>Model-based spectra of leaf reflectance at <span class="html-italic">F<sub>S</sub> </span>= 0 (<b>a</b>), <span class="html-italic">F<sub>S</sub> </span>= 0.075 (<b>b</b>), <span class="html-italic">F<sub>S</sub> </span>= 0.15 (<b>c</b>), and <span class="html-italic">F<sub>S</sub> </span>= 0.225 (<b>d</b>) and the experimental spectrum (from <a href="#plants-13-03258-f005" class="html-fig">Figure 5</a>). Other parameters of the model were the same as the parameters that were used for the simulation of the spectrum in <a href="#plants-13-03258-f007" class="html-fig">Figure 7</a>. R<sup>2</sup> and RMSE are, respectively, the determination coefficient and root mean square error between the experimental and model-based spectra.</p> Full article ">Figure 9
<p>Experimental concentrations of chlorophyll a, chlorophyll b, and carotenoids (<b>a</b>) and thicknesses of palisade and spongy mesophyll (<b>b</b>) in second mature leaves of pea plants, which were cultivated for 16 days under low and moderate light intensity (<span class="html-italic">n</span> = 6). *, the value is significantly different from this value in plants cultivated under moderate light intensity.</p> Full article ">Figure 10
<p>Experimental (<b>a</b>) and model-based (<b>b</b>) spectra of leaf reflectance in pea plants, which were cultivated for 16 days under low and moderate light intensity (<span class="html-italic">n</span> = 6 for experiments). The average experimental reflectance spectrum (<span class="html-italic">n</span> = 6) measured in the second mature leaf of pea plants (PolyPen RP 410) is shown. Standard errors are not shown because they are small. In variant “Moderate light intensity”, parameters of the model were the same as the parameters that were used for the simulation of the spectrum in <a href="#plants-13-03258-f007" class="html-fig">Figure 7</a>. In variant “Low light intensity”, <span class="html-italic">h</span> = 25.2 μm and <span class="html-italic">l</span> = 47.8 μm (see <a href="#plants-13-03258-f009" class="html-fig">Figure 9</a>b); other parameters were not changed. R<sup>2</sup> between experimental and model-based dependences were 0.989 (variant “moderate light intensity”) and 0.982 (variant “low light intensity”).</p> Full article ">Figure 11
<p>Spectra of leaf reflectance calculated at <span class="html-italic">h</span> = 45 μm, <span class="html-italic">h</span> = 35 μm, and <span class="html-italic">h</span> = 25 μm (<b>a</b>) and at <span class="html-italic">l</span> = 80 μm, <span class="html-italic">l</span> = 60 μm, and <span class="html-italic">l</span> = 40 μm (<b>b</b>). Other parameters of the model were the same as the parameters that were used for the simulation of the spectrum in <a href="#plants-13-03258-f008" class="html-fig">Figure 8</a>c.</p> Full article ">
<p>The scheme of light flows in the model of reflectance and transmission in the leaf of a dicot plant. Black continuous lines show forward light flows. Black dotted lines show backward light flows. Orange lines show transformation between light flows. <span class="html-italic">I<sub>C</sub></span> is the forward collimated light, <span class="html-italic">I<sub>S</sub></span> is the forward scattered light, <span class="html-italic">J<sub>C</sub></span> is the backward collimated light, and <span class="html-italic">J<sub>S</sub></span> is the backward scattered light. <span class="html-italic">I<sub>0</sub></span> and <span class="html-italic">J<sub>0</sub></span> are intensities of the forward and backward collimated light directed to adaxial and abaxial leaf surfaces, respectively (the incident light). <span class="html-italic">J<sub>C</sub><sup>RO</sup></span> and <span class="html-italic">I<sub>C</sub><sup>RO</sup></span> are intensities of the collimated light reflecting from adaxial and abaxial leaf surfaces in air. <span class="html-italic">J<sub>S</sub><sup>RO</sup></span> and <span class="html-italic">I<sub>S</sub><sup>RO</sup></span> are intensities of the scattered light reflecting from adaxial and abaxial leaf surfaces in air. <span class="html-italic">J<sub>C</sub><sup>T</sup></span> and <span class="html-italic">I<sub>C</sub><sup>T</sup></span> are intensities of the collimated light transferring from leaf to air across adaxial and abaxial surfaces. <span class="html-italic">J<sub>S</sub><sup>T</sup></span> and <span class="html-italic">I<sub>S</sub><sup>T</sup></span> are intensities of the scattered light transferring from leaf to air across adaxial and abaxial surfaces.</p> Full article ">Figure 2
<p>Image of pea leaf cross-section (<b>a</b>) and average thickness of palisade (<span class="html-italic">h</span>) and spongy (<span class="html-italic">l</span>) mesophyll (<span class="html-italic">n</span> = 6) (<b>b</b>). The second mature leaf was used. Pea plants were cultivated for 16 days under the moderate light intensity.</p> Full article ">Figure 3
<p>Spectra of specific light absorption coefficients for chlorophyll a, chlorophyll b, and carotenoids (on an example of β-carotene) (<b>a</b>) and average concentrations of these pigments in pea leaves (<span class="html-italic">n</span> = 6) (<b>b</b>). The spectra of light absorption were constructed on the basis of [<a href="#B58-plants-13-03258" class="html-bibr">58</a>]. The pigment concentrations were experimentally measured. The second mature leaf was used. Pea plants were cultivated for 16 days under moderate light intensity.</p> Full article ">Figure 4
<p>Spectra of leaf reflectance calculated on basis of the developed model with different quantities of the iterations (<span class="html-italic">N</span>). The leaf reflectance was calculated as a ratio of the sum of all components of backward collimated and backward scattered light transferring through the adaxial leaf surface (<span class="html-italic">J<sub>out</sub></span>) to the intensity of incident light (<span class="html-italic">I</span><sub>0</sub>). The influence of <span class="html-italic">N</span> on <span class="html-italic">J<sub>out</sub></span> was calculated in accordance with Equation (58). The following parameters were used (see <a href="#sec2dot1-plants-13-03258" class="html-sec">Section 2.1</a> for details): <span class="html-italic">β</span><sub>O1</sub> = 35° (in accordance with angle of the leaf illumination by PolyPen RP 410), <span class="html-italic">I<sub>0</sub> </span>= 1000 μmol m<sup>−2</sup>s<sup>−1</sup> and <span class="html-italic">J</span><sub>0</sub> = 0 μmol m<sup>−2</sup>s<sup>−1</sup> (assumed), <span class="html-italic">F<sub>S</sub> </span>= 0 (assumed), <span class="html-italic">n<sub>I</sub> </span>= 1.415 [<a href="#B50-plants-13-03258" class="html-bibr">50</a>], <span class="html-italic">f</span> = 0.5 (assumed), <span class="html-italic">h</span> = 35.5 μm and <span class="html-italic">l</span> = 58.6 μm (<a href="#plants-13-03258-f002" class="html-fig">Figure 2</a>b), <math display="inline"><semantics> <mrow> <msub> <mrow> <mi>s</mi> </mrow> <mrow> <mi>P</mi> </mrow> </msub> <mo>=</mo> </mrow> </semantics></math> 5 cm<sup>−1</sup> and <math display="inline"><semantics> <mrow> <msub> <mrow> <mi>s</mi> </mrow> <mrow> <mi>S</mi> <mi>p</mi> </mrow> </msub> </mrow> </semantics></math> = 1000 cm<sup>−1</sup> [<a href="#B49-plants-13-03258" class="html-bibr">49</a>], C<sub>ChA</sub> = 2.77 mg cm<sup>−3</sup>, C<sub>ChB</sub> = 1.69 mg cm<sup>−3</sup>, and C<sub>Car</sub> = 0.94 mg cm<sup>−3</sup> corresponded to average experimental concentrations of these pigments (1.39, 0.85, and 0.47 mg cm<sup>−3</sup>, respectively, <a href="#plants-13-03258-f003" class="html-fig">Figure 3</a>b) at <span class="html-italic">N<sub>Sp/P</sub> </span>= 0.2 [<a href="#B49-plants-13-03258" class="html-bibr">49</a>]); <math display="inline"><semantics> <mrow> <msub> <mrow> <mi>a</mi> </mrow> <mrow> <mi>C</mi> <mi>h</mi> <mi>A</mi> </mrow> </msub> <mfenced separators="|"> <mrow> <mi>λ</mi> </mrow> </mfenced> </mrow> </semantics></math>, <math display="inline"><semantics> <mrow> <msub> <mrow> <mi>a</mi> </mrow> <mrow> <mi>C</mi> <mi>h</mi> <mi>B</mi> </mrow> </msub> <mfenced separators="|"> <mrow> <mi>λ</mi> </mrow> </mfenced> </mrow> </semantics></math>, and <math display="inline"><semantics> <mrow> <msub> <mrow> <mi>a</mi> </mrow> <mrow> <mi>C</mi> <mi>a</mi> <mi>r</mi> </mrow> </msub> <mfenced separators="|"> <mrow> <mi>λ</mi> </mrow> </mfenced> </mrow> </semantics></math> are shown in <a href="#plants-13-03258-f003" class="html-fig">Figure 3</a>a.</p> Full article ">Figure 5
<p>Experimental and model-based spectra of leaf reflectance. The average experimental reflectance spectrum (<span class="html-italic">n</span> = 6) measured in the second mature leaf of pea plants (PolyPen RP 410) is shown. Standard errors are not shown because they are small. Pea plants were cultivated for 16 days under the moderate light intensity. Parameters of the model are shown in <a href="#plants-13-03258-f004" class="html-fig">Figure 4</a>; <span class="html-italic">N</span> = 6 was used for the analysis. R<sup>2</sup> and RMSE are the determination coefficient and root mean square error between the experimental and model-based spectra.</p> Full article ">Figure 6
<p>Model-based spectra of leaf reflectance at <math display="inline"><semantics> <mrow> <msub> <mrow> <mi>s</mi> </mrow> <mrow> <mi>S</mi> <mi>p</mi> </mrow> </msub> <mo>=</mo> </mrow> </semantics></math> 1000 cm<sup>−1</sup> (<b>a</b>), <math display="inline"><semantics> <mrow> <msub> <mrow> <mi>s</mi> </mrow> <mrow> <mi>S</mi> <mi>p</mi> </mrow> </msub> <mo>=</mo> </mrow> </semantics></math> 800 cm<sup>−1</sup> (<b>b</b>), <math display="inline"><semantics> <mrow> <msub> <mrow> <mi>s</mi> </mrow> <mrow> <mi>S</mi> <mi>p</mi> </mrow> </msub> <mo>=</mo> </mrow> </semantics></math> 600 cm<sup>−1</sup> (<b>c</b>), and <math display="inline"><semantics> <mrow> <msub> <mrow> <mi>s</mi> </mrow> <mrow> <mi>S</mi> <mi>p</mi> </mrow> </msub> <mo>=</mo> </mrow> </semantics></math> 400 cm<sup>−1</sup> (<b>d</b>) and the experimental spectrum (from <a href="#plants-13-03258-f005" class="html-fig">Figure 5</a>). Other parameters of the model were the same as the parameters that were used for the simulation of the spectrum in <a href="#plants-13-03258-f005" class="html-fig">Figure 5</a>. R<sup>2</sup> and RMSE are, respectively, the determination coefficient and root mean square error between the experimental and model-based spectra.</p> Full article ">Figure 7
<p>(<b>a</b>) Model-based spectrum of leaf reflectance at C<sub>ChA</sub> = 3.19 mg cm<sup>−3</sup> and C<sub>ChB</sub> = 2.09 mg cm<sup>−3</sup> and the experimental spectrum (from <a href="#plants-13-03258-f005" class="html-fig">Figure 5</a>). Other parameters of the model were the same as the parameters that were used for the simulation of the spectrum in <a href="#plants-13-03258-f006" class="html-fig">Figure 6</a>c. R<sup>2</sup> and RMSE are, respectively, the determination coefficient and root mean square error between the experimental and model-based spectra. (<b>b</b>) Average concentrations of chlorophyll a and b, which corresponded to C<sub>ChA</sub> = 3.19 mg cm<sup>−3</sup> and C<sub>ChB</sub> = 2.09 mg cm<sup>−3</sup>, and their experimental concentrations in pea leaves (form <a href="#plants-13-03258-f003" class="html-fig">Figure 3</a>).</p> Full article ">Figure 8
<p>Model-based spectra of leaf reflectance at <span class="html-italic">F<sub>S</sub> </span>= 0 (<b>a</b>), <span class="html-italic">F<sub>S</sub> </span>= 0.075 (<b>b</b>), <span class="html-italic">F<sub>S</sub> </span>= 0.15 (<b>c</b>), and <span class="html-italic">F<sub>S</sub> </span>= 0.225 (<b>d</b>) and the experimental spectrum (from <a href="#plants-13-03258-f005" class="html-fig">Figure 5</a>). Other parameters of the model were the same as the parameters that were used for the simulation of the spectrum in <a href="#plants-13-03258-f007" class="html-fig">Figure 7</a>. R<sup>2</sup> and RMSE are, respectively, the determination coefficient and root mean square error between the experimental and model-based spectra.</p> Full article ">Figure 9
<p>Experimental concentrations of chlorophyll a, chlorophyll b, and carotenoids (<b>a</b>) and thicknesses of palisade and spongy mesophyll (<b>b</b>) in second mature leaves of pea plants, which were cultivated for 16 days under low and moderate light intensity (<span class="html-italic">n</span> = 6). *, the value is significantly different from this value in plants cultivated under moderate light intensity.</p> Full article ">Figure 10
<p>Experimental (<b>a</b>) and model-based (<b>b</b>) spectra of leaf reflectance in pea plants, which were cultivated for 16 days under low and moderate light intensity (<span class="html-italic">n</span> = 6 for experiments). The average experimental reflectance spectrum (<span class="html-italic">n</span> = 6) measured in the second mature leaf of pea plants (PolyPen RP 410) is shown. Standard errors are not shown because they are small. In variant “Moderate light intensity”, parameters of the model were the same as the parameters that were used for the simulation of the spectrum in <a href="#plants-13-03258-f007" class="html-fig">Figure 7</a>. In variant “Low light intensity”, <span class="html-italic">h</span> = 25.2 μm and <span class="html-italic">l</span> = 47.8 μm (see <a href="#plants-13-03258-f009" class="html-fig">Figure 9</a>b); other parameters were not changed. R<sup>2</sup> between experimental and model-based dependences were 0.989 (variant “moderate light intensity”) and 0.982 (variant “low light intensity”).</p> Full article ">Figure 11
<p>Spectra of leaf reflectance calculated at <span class="html-italic">h</span> = 45 μm, <span class="html-italic">h</span> = 35 μm, and <span class="html-italic">h</span> = 25 μm (<b>a</b>) and at <span class="html-italic">l</span> = 80 μm, <span class="html-italic">l</span> = 60 μm, and <span class="html-italic">l</span> = 40 μm (<b>b</b>). Other parameters of the model were the same as the parameters that were used for the simulation of the spectrum in <a href="#plants-13-03258-f008" class="html-fig">Figure 8</a>c.</p> Full article ">
Open AccessArticle
Effects of Sugarcane/Peanut Intercropping on Root Exudates and Rhizosphere Soil Nutrient
by
Xiumei Tang, Lulu Liao, Haining Wu, Jun Xiong, Zhong Li, Zhipeng Huang, Liangqiong He, Jing Jiang, Ruichun Zhong, Zhuqiang Han and Ronghua Tang
Plants 2024, 13(22), 3257; https://doi.org/10.3390/plants13223257 - 20 Nov 2024
Abstract
Intercropping can enable more efficient resource use and increase yield. Most current studies focus on the correlation between soil nutrients and crop yield under intercropping conditions. However, the mechanisms related to root exudates and soil nutrients remain unclear. Therefore, this study explored the
[...] Read more.
Intercropping can enable more efficient resource use and increase yield. Most current studies focus on the correlation between soil nutrients and crop yield under intercropping conditions. However, the mechanisms related to root exudates and soil nutrients remain unclear. Therefore, this study explored the correlation between rhizosphere soil nutrients and root exudates in sugarcane/peanut intercropping. Root extracts, root exudates, rhizosphere soil enzyme activities, and soil nutrients were analyzed and compared in monocultured and intercropped peanut and sugarcane at different growth stages. The root metabolites were annotated using the Kyoto Encyclopedia of Genes and Genomes pathways to further identify the connection between soil nutrients and root exudates. The effects of intercropping differed in peanut and sugarcane at different growth stages, and the difference between podding and pod-filling stages was significant. Intercropping generally had a great effect on peanut; it not only significantly increased the organic acid, soluble sugars, and phenolic acids in root exudates and extracts from peanuts, but also significantly increased rhizosphere soil enzyme activities and soil nutrient levels. Intercropping peanuts promoted fumaric acid secretion from roots and significantly affected the metabolic pathways of alanine, aspartate, and glutamate. Sugarcane/peanut intercropping can increase root exudates and effectively improve soil nutrients. The changes in soil nutrients are closely related to the effects of fumaric acid on alanine, aspartate, and glutamate metabolism.
Full article
(This article belongs to the Section Plant–Soil Interactions)
►▼
Show Figures
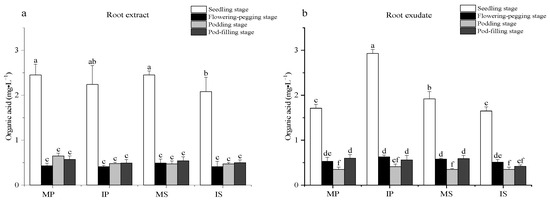
Figure 1
Figure 1
<p>Effects of sugarcane/peanut intercropping on organic acids. (<b>a</b>) represents the change of organic acids in root extract under intercropping conditions, (<b>b</b>) represents the change of organic acids in root exudate under intercropping. Note: Different letters in the bar chart indicate significant differences (<span class="html-italic">p</span> < 0.05), the same below.</p> Full article ">Figure 2
<p>Effect of sugarcane/peanut intercropping on soluble sugar content. (<b>a</b>) shows the change of soluble sugar in root extract under intercropping conditions, (<b>b</b>) shows the change of soluble sugar in root exudate under intercropping.</p> Full article ">Figure 3
<p>Effect of sugarcane/peanut intercropping on amino acids. (<b>a</b>) shows the change of amino acid in root extract under intercropping conditions, (<b>b</b>) shows the change of amino acid in root exudate under intercropping.</p> Full article ">Figure 4
<p>Effects of sugarcane/peanut intercropping on phenolic acids. (<b>a</b>) shows the change of phenolic acid in root extract under intercropping conditions, (<b>b</b>) shows the change of phenolic acid in root exudate under intercropping.</p> Full article ">Figure 5
<p>Effects of sugarcane/peanut intercropping on differential metabolites. Note: (<b>a</b>) shows the metabolites detected in monoculture peanut, intercrop peanut, monoculture sugarcane and intercrop sugarcane. (<b>b</b>) shows the trend and degree of difference of metabolites between MP and IP, (<b>c</b>) shows the trend and degree of difference of metabolites between MS and IS. In (<b>b</b>,<b>c</b>), the horizontal coordinate is the converted Z-score value of the relative content of metabolites in the sale, the vertical coordinate is the name of metabolites, and the color of the points represents different groups.</p> Full article ">Figure 6
<p>Effects of sugarcane/peanut intercropping on metabolic pathways. (<b>a</b>) shows enrichment of MP and IP metabolic pathways, (<b>b</b>) shows enrichment of MS and IS metabolic pathways. Note: the horizontal coordinate represents the Impact value that is enriched into different metabolic pathways, the vertical coordinate represents the metabolic pathway, and the number represents the corresponding number of metabolites on the pathway. Impact is the influence value of metabolic pathway, and the larger the impact of differential metabolites on the target pathway is. Color is correlated with the <span class="html-italic">p</span>-value, the redder the color, the smaller the <span class="html-italic">p</span>-value, the bluer the color, the larger the <span class="html-italic">p</span>-value, and the smaller the <span class="html-italic">p</span>-value, which means that the different metabolites have a more significant impact on this pathway.</p> Full article ">Figure 7
<p>Correlation analysis of different metabolism and rhizosphere soil nutrients and enzyme activities of monoculture peanut and intercropping peanut.</p> Full article ">
<p>Effects of sugarcane/peanut intercropping on organic acids. (<b>a</b>) represents the change of organic acids in root extract under intercropping conditions, (<b>b</b>) represents the change of organic acids in root exudate under intercropping. Note: Different letters in the bar chart indicate significant differences (<span class="html-italic">p</span> < 0.05), the same below.</p> Full article ">Figure 2
<p>Effect of sugarcane/peanut intercropping on soluble sugar content. (<b>a</b>) shows the change of soluble sugar in root extract under intercropping conditions, (<b>b</b>) shows the change of soluble sugar in root exudate under intercropping.</p> Full article ">Figure 3
<p>Effect of sugarcane/peanut intercropping on amino acids. (<b>a</b>) shows the change of amino acid in root extract under intercropping conditions, (<b>b</b>) shows the change of amino acid in root exudate under intercropping.</p> Full article ">Figure 4
<p>Effects of sugarcane/peanut intercropping on phenolic acids. (<b>a</b>) shows the change of phenolic acid in root extract under intercropping conditions, (<b>b</b>) shows the change of phenolic acid in root exudate under intercropping.</p> Full article ">Figure 5
<p>Effects of sugarcane/peanut intercropping on differential metabolites. Note: (<b>a</b>) shows the metabolites detected in monoculture peanut, intercrop peanut, monoculture sugarcane and intercrop sugarcane. (<b>b</b>) shows the trend and degree of difference of metabolites between MP and IP, (<b>c</b>) shows the trend and degree of difference of metabolites between MS and IS. In (<b>b</b>,<b>c</b>), the horizontal coordinate is the converted Z-score value of the relative content of metabolites in the sale, the vertical coordinate is the name of metabolites, and the color of the points represents different groups.</p> Full article ">Figure 6
<p>Effects of sugarcane/peanut intercropping on metabolic pathways. (<b>a</b>) shows enrichment of MP and IP metabolic pathways, (<b>b</b>) shows enrichment of MS and IS metabolic pathways. Note: the horizontal coordinate represents the Impact value that is enriched into different metabolic pathways, the vertical coordinate represents the metabolic pathway, and the number represents the corresponding number of metabolites on the pathway. Impact is the influence value of metabolic pathway, and the larger the impact of differential metabolites on the target pathway is. Color is correlated with the <span class="html-italic">p</span>-value, the redder the color, the smaller the <span class="html-italic">p</span>-value, the bluer the color, the larger the <span class="html-italic">p</span>-value, and the smaller the <span class="html-italic">p</span>-value, which means that the different metabolites have a more significant impact on this pathway.</p> Full article ">Figure 7
<p>Correlation analysis of different metabolism and rhizosphere soil nutrients and enzyme activities of monoculture peanut and intercropping peanut.</p> Full article ">
Open AccessArticle
Diversity Patterns of Epiphytic Orchids Along Elevation in the Mountains of Western Nepal
by
Manisha Awasthi, Santosh Thapa, Bandana Awasthi, Chae Ryeong Lim, Young Han You and Ki Wha Chung
Plants 2024, 13(22), 3256; https://doi.org/10.3390/plants13223256 - 20 Nov 2024
Abstract
The biodiversity and distribution of epiphytic orchids are strongly influenced by their relationship with host plants, and environmental variables like elevation, slope, and local climate are key factors in determining the abundance and diversity of these orchids. The aim of this study was
[...] Read more.
The biodiversity and distribution of epiphytic orchids are strongly influenced by their relationship with host plants, and environmental variables like elevation, slope, and local climate are key factors in determining the abundance and diversity of these orchids. The aim of this study was to examine the richness of orchid species at different elevations within the research area of Nepal. Sampling was conducted at elevations ranging from 1300 m to 2800 m above sea level, using a systematic sampling technique known as belt transects. Six circular plots with a diameter of 5.6 m were established in a horizontal transect at each site, spaced at least 50 m apart, with 100 elevation interval. The analysis revealed a hump-shaped relationship between orchid species richness and elevation, with the highest species richness observed at altitude of 2100–2200 m. The abundance of orchids was significantly correlated with host characteristics, including habit (shrub/tree), bark texture, nature (deciduous/evergreen), and physical factors. This study underscores the significant contribution of host characteristics and environmental factors in explaining the diversity of epiphytic orchid species along the elevation of the Himalayas.
Full article
(This article belongs to the Special Issue Orchid Conservation and Biodiversity)
►▼
Show Figures
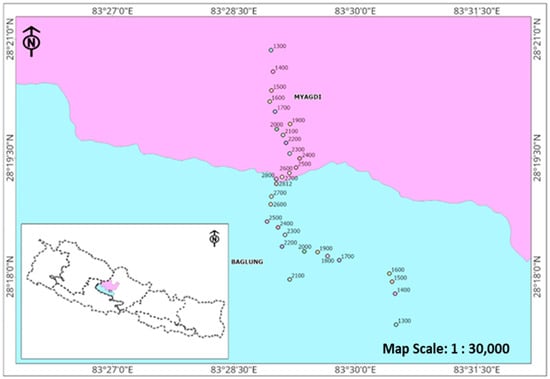
Figure 1
Figure 1
<p>Map of Nepal showing study areas with study sites along the elevation. Examined plots are indicated with altitude (Baglung in blue and Myagdi in pink).</p> Full article ">Figure 2
<p>Some epiphytic orchid species observed in the Tinchule Mountain, Nepal. (<b>A</b>) <span class="html-italic">Pleione praecox</span>: found mainly on <span class="html-italic">Rhododendron arboreum</span>, (<b>B</b>) <span class="html-italic">Dendrobium amoenum</span>: recorded across all host layers, primarily in L3, L4, and L5 on <span class="html-italic">Alnus nepalensis</span>, (<b>C</b>) <span class="html-italic">Rhynchostylis retusa</span>: threatened due to its ornamental use, it grows mostly on deciduous and semi-deciduous trees like <span class="html-italic">Engelhardia spicata</span>, (<b>D</b>) <span class="html-italic">Dendrobium densiflorum</span>: abundant in the study area, especially in <span class="html-italic">Castonopsis</span> forests, (<b>E</b>) <span class="html-italic">Pholidota pallida</span>: found mainly on <span class="html-italic">Lyonia ovalifolia</span>, which has a highly rugose bark, and (<b>F</b>) <span class="html-italic">Cymbidium erythraeum</span>: prefers mossy trunks, particularly <span class="html-italic">Quercus semecarpifolia</span>.</p> Full article ">Figure 3
<p>Species richness along the elevation of the study areas. Each dot indicates the observed number of orchid species in each examined plot. Horizontal and vertical lines indicate the mean richness values and standard deviations, respectively.</p> Full article ">Figure 4
<p>Relative frequencies of epiphytic orchids with host families.</p> Full article ">Figure 5
<p>Relations between numbers of epiphytic orchid species with host properties and land uses: (<b>A</b>) diameter at breast height (DBH) of the host tree (cm), (<b>B</b>) height of the host species (m), (<b>C</b>) types of host species, (<b>D</b>) bark textures of the host species, (<b>E</b>) vertical layers of the host species, and (<b>F</b>) land uses where host species are grown (* <span class="html-italic">p</span> < 0.05; ** <span class="html-italic">p</span> < 0.01; and *** <span class="html-italic">p</span> < 0.001).</p> Full article ">Figure 6
<p>Canonical correspondence analysis showing the association between different epiphytic orchid species composition and predicted variables of elevation, diameter at breast height (DBH), height, and rugosity. The red lines show the predicted variables, where the blue triangles indicate the host plot.</p> Full article ">
<p>Map of Nepal showing study areas with study sites along the elevation. Examined plots are indicated with altitude (Baglung in blue and Myagdi in pink).</p> Full article ">Figure 2
<p>Some epiphytic orchid species observed in the Tinchule Mountain, Nepal. (<b>A</b>) <span class="html-italic">Pleione praecox</span>: found mainly on <span class="html-italic">Rhododendron arboreum</span>, (<b>B</b>) <span class="html-italic">Dendrobium amoenum</span>: recorded across all host layers, primarily in L3, L4, and L5 on <span class="html-italic">Alnus nepalensis</span>, (<b>C</b>) <span class="html-italic">Rhynchostylis retusa</span>: threatened due to its ornamental use, it grows mostly on deciduous and semi-deciduous trees like <span class="html-italic">Engelhardia spicata</span>, (<b>D</b>) <span class="html-italic">Dendrobium densiflorum</span>: abundant in the study area, especially in <span class="html-italic">Castonopsis</span> forests, (<b>E</b>) <span class="html-italic">Pholidota pallida</span>: found mainly on <span class="html-italic">Lyonia ovalifolia</span>, which has a highly rugose bark, and (<b>F</b>) <span class="html-italic">Cymbidium erythraeum</span>: prefers mossy trunks, particularly <span class="html-italic">Quercus semecarpifolia</span>.</p> Full article ">Figure 3
<p>Species richness along the elevation of the study areas. Each dot indicates the observed number of orchid species in each examined plot. Horizontal and vertical lines indicate the mean richness values and standard deviations, respectively.</p> Full article ">Figure 4
<p>Relative frequencies of epiphytic orchids with host families.</p> Full article ">Figure 5
<p>Relations between numbers of epiphytic orchid species with host properties and land uses: (<b>A</b>) diameter at breast height (DBH) of the host tree (cm), (<b>B</b>) height of the host species (m), (<b>C</b>) types of host species, (<b>D</b>) bark textures of the host species, (<b>E</b>) vertical layers of the host species, and (<b>F</b>) land uses where host species are grown (* <span class="html-italic">p</span> < 0.05; ** <span class="html-italic">p</span> < 0.01; and *** <span class="html-italic">p</span> < 0.001).</p> Full article ">Figure 6
<p>Canonical correspondence analysis showing the association between different epiphytic orchid species composition and predicted variables of elevation, diameter at breast height (DBH), height, and rugosity. The red lines show the predicted variables, where the blue triangles indicate the host plot.</p> Full article ">
Open AccessReview
Recent Advances in Postharvest Application of Exogenous Phytohormones for Quality Preservation of Fruits and Vegetables
by
Sbulelo Mwelase, Jerry O. Adeyemi and Olaniyi A. Fawole
Plants 2024, 13(22), 3255; https://doi.org/10.3390/plants13223255 - 20 Nov 2024
Abstract
The increasing global population has heightened the demand for food, leading to escalated food production and, consequently, the generation of significant food waste. Factors such as rapid ripening, susceptibility to physiological disorders, and vulnerability to microbial attacks have been implicated as contributing to
[...] Read more.
The increasing global population has heightened the demand for food, leading to escalated food production and, consequently, the generation of significant food waste. Factors such as rapid ripening, susceptibility to physiological disorders, and vulnerability to microbial attacks have been implicated as contributing to the accelerated senescence associated with food waste generation. Fruits and vegetables, characterized by their high perishability, account for approximately half of all food waste produced, rendering them a major area of concern. Various postharvest technologies have thus been employed, including the application of phytohormone treatments, to safeguard and extend the storability of highly perishable food products. This review, therefore, explores the physicochemical properties and biological aspects of phytohormones that render them suitable for food preservation. Furthermore, this review examines the effects of externally applied phytohormones on the postharvest physiology and quality attributes of fresh produce. Finally, the review investigates the mechanisms by which exogenous phytohormones preserve food quality and discusses the associated limitations and safety considerations related to the use of these compounds in food applications.
Full article
(This article belongs to the Special Issue Postharvest Quality and Physiology of Vegetables and Fruits)
►▼
Show Figures
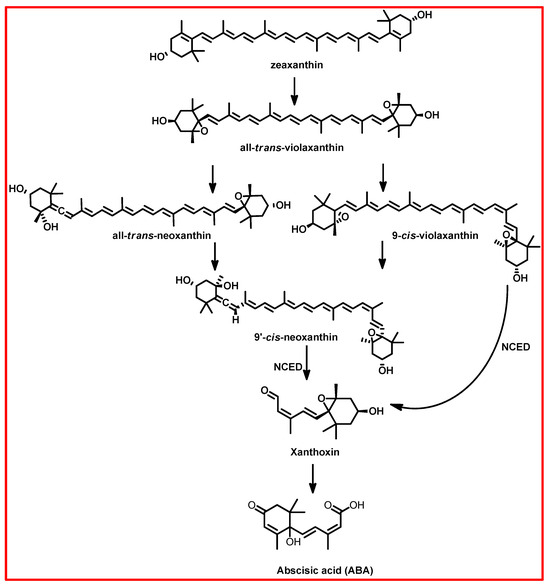
Figure 1
Figure 1
<p>Abscisic acid (ABA) biosynthesis in plants. Adapted from [<a href="#B73-plants-13-03255" class="html-bibr">73</a>].</p> Full article ">Figure 2
<p>Ethylene biosynthesis pathway, adapted from [<a href="#B116-plants-13-03255" class="html-bibr">116</a>].</p> Full article ">Figure 3
<p>Major pathways for Trp-dependent and Trp-independent IAA synthesis [<a href="#B125-plants-13-03255" class="html-bibr">125</a>].</p> Full article ">Figure 4
<p>The biosynthesis pathway of gibberellins in plants [<a href="#B130-plants-13-03255" class="html-bibr">130</a>].</p> Full article ">Figure 5
<p>The biosynthesis pathway of cytokinins in plants [<a href="#B142-plants-13-03255" class="html-bibr">142</a>].</p> Full article ">Figure 6
<p>The biosynthesis pathway of jasmonates in plants [<a href="#B150-plants-13-03255" class="html-bibr">150</a>].</p> Full article ">Figure 7
<p>The biosynthesis pathway of salicylic acid [<a href="#B158-plants-13-03255" class="html-bibr">158</a>]. AAO, aldehyde oxidase; BA2H, benzoic acid 2-hydroxylase; CM, chorismate mutase; ICS, isochorismate synthase; IPL, isochorismate pyruvate lyase; PAL, phenylalanine ammonia-lyase.</p> Full article ">Figure 8
<p>The biosynthesis pathway of brassinosteroids [<a href="#B167-plants-13-03255" class="html-bibr">167</a>,<a href="#B170-plants-13-03255" class="html-bibr">170</a>]. Red arrows represent a predominant 8-step brassinosteroid biosynthetic pathway using campestanol-independent subroutes. Blue arrows represent a 10-step campestanol-dependent brassinosteroid biosynthetic pathway. CPD, constitutive photomorphogenesis and dwarfism; DET2, de-etiolated 2; DWF4, dwarf 4; ROT3/CYP90D1, rotundifolia 3/cytochrome P450 90D1.</p> Full article ">Figure 9
<p>Structure of naturally occurring (<b>A</b>) strigol and synthetic analogue (<b>B</b>) GR24 [<a href="#B172-plants-13-03255" class="html-bibr">172</a>].</p> Full article ">Figure 10
<p>Biosynthesis of strigolactones in plants [<a href="#B172-plants-13-03255" class="html-bibr">172</a>]. carotenoid cleavage dioxygenase—CCD.</p> Full article ">Figure 11
<p>The biosynthesis of melatonin under normal growth conditions (<b>A</b>) and under serotonin boost conditions (<b>B</b>). Catalytic efficiency (CE) means the Kcat/Km values (mmol/L min<sup>−1</sup>), which were measured at 37 °C except for SNAT, which was measured at 30°C. *CE indicates values measured at 55 °C. TDC, tryptophan decarboxylase; T5H, tryptamine 5-hydroxylase; SNAT, serotonin N-acetyltransferase; COMT, caffeic acid O-methyltransferase; ASMT, N-acetylserotonin methyltransferase [<a href="#B195-plants-13-03255" class="html-bibr">195</a>].</p> Full article ">
<p>Abscisic acid (ABA) biosynthesis in plants. Adapted from [<a href="#B73-plants-13-03255" class="html-bibr">73</a>].</p> Full article ">Figure 2
<p>Ethylene biosynthesis pathway, adapted from [<a href="#B116-plants-13-03255" class="html-bibr">116</a>].</p> Full article ">Figure 3
<p>Major pathways for Trp-dependent and Trp-independent IAA synthesis [<a href="#B125-plants-13-03255" class="html-bibr">125</a>].</p> Full article ">Figure 4
<p>The biosynthesis pathway of gibberellins in plants [<a href="#B130-plants-13-03255" class="html-bibr">130</a>].</p> Full article ">Figure 5
<p>The biosynthesis pathway of cytokinins in plants [<a href="#B142-plants-13-03255" class="html-bibr">142</a>].</p> Full article ">Figure 6
<p>The biosynthesis pathway of jasmonates in plants [<a href="#B150-plants-13-03255" class="html-bibr">150</a>].</p> Full article ">Figure 7
<p>The biosynthesis pathway of salicylic acid [<a href="#B158-plants-13-03255" class="html-bibr">158</a>]. AAO, aldehyde oxidase; BA2H, benzoic acid 2-hydroxylase; CM, chorismate mutase; ICS, isochorismate synthase; IPL, isochorismate pyruvate lyase; PAL, phenylalanine ammonia-lyase.</p> Full article ">Figure 8
<p>The biosynthesis pathway of brassinosteroids [<a href="#B167-plants-13-03255" class="html-bibr">167</a>,<a href="#B170-plants-13-03255" class="html-bibr">170</a>]. Red arrows represent a predominant 8-step brassinosteroid biosynthetic pathway using campestanol-independent subroutes. Blue arrows represent a 10-step campestanol-dependent brassinosteroid biosynthetic pathway. CPD, constitutive photomorphogenesis and dwarfism; DET2, de-etiolated 2; DWF4, dwarf 4; ROT3/CYP90D1, rotundifolia 3/cytochrome P450 90D1.</p> Full article ">Figure 9
<p>Structure of naturally occurring (<b>A</b>) strigol and synthetic analogue (<b>B</b>) GR24 [<a href="#B172-plants-13-03255" class="html-bibr">172</a>].</p> Full article ">Figure 10
<p>Biosynthesis of strigolactones in plants [<a href="#B172-plants-13-03255" class="html-bibr">172</a>]. carotenoid cleavage dioxygenase—CCD.</p> Full article ">Figure 11
<p>The biosynthesis of melatonin under normal growth conditions (<b>A</b>) and under serotonin boost conditions (<b>B</b>). Catalytic efficiency (CE) means the Kcat/Km values (mmol/L min<sup>−1</sup>), which were measured at 37 °C except for SNAT, which was measured at 30°C. *CE indicates values measured at 55 °C. TDC, tryptophan decarboxylase; T5H, tryptamine 5-hydroxylase; SNAT, serotonin N-acetyltransferase; COMT, caffeic acid O-methyltransferase; ASMT, N-acetylserotonin methyltransferase [<a href="#B195-plants-13-03255" class="html-bibr">195</a>].</p> Full article ">
Open AccessArticle
Contrasting Weather and Stocking Effects on Eucalyptus Initial Coppice Response in Brazil
by
Pietro Gragnolati Fernandes, Clayton Alcarde Alvares, Túlio Barroso Queiroz, Pedro Vitor Pimenta, Jarbas Silva Borges, James Stahl, Flávio Teixeira Mendes, Amanda Souza, Gustavo Matheus Silva, Gualter Guenther Costa da Silva, Sara Bezerra Bandeira Milhomem, Rosilvam Ramos de Sousa and Rodrigo Eiji Hakamada
Plants 2024, 13(22), 3254; https://doi.org/10.3390/plants13223254 - 20 Nov 2024
Abstract
In Eucalyptus plantations, coppice rotations often yield less than initial rotations. The TECHS project (Tolerance of Eucalyptus Clones to Hydric, Thermal and Biotic Stresses) studied short rotation coppicing across a 3000 km gradient. The main objective of this work was to compare the
[...] Read more.
In Eucalyptus plantations, coppice rotations often yield less than initial rotations. The TECHS project (Tolerance of Eucalyptus Clones to Hydric, Thermal and Biotic Stresses) studied short rotation coppicing across a 3000 km gradient. The main objective of this work was to compare the survival, sprouting, and initial growth of Eucalyptus clones managed and to examine factors that might influence the productivity of the coppice rotation: climate, genotypes, and stocking. Eight of the TECHS sites spread from latitudes 6° S to 30° S were included in the coppice study, with 17 genotypes at each site. The initial rotation had been planted at a 3 m × 3 m spacing and also in a spacing trial at densities from 500 to 3500 trees ha−1. Six months after harvesting the initial Eucalyptus rotation, average survival was 88%, with tropical clones showing over twice the sprouting biomass (6.7 vs. 2.9 Mg ha−1) and four times the woody biomass compared to subtropical clones (4.7 vs. 1.1 Mg ha−1). Greater initial water deficits had stronger sprouting and growth. Clones with higher belowground carbon allocation in the initial rotation performed better in coppicing, and precipitation became more influential after 12 months. Drought and spacing trials significantly affected growth.
Full article
(This article belongs to the Special Issue Morphological, Physiological and Carbon Balance Response of Trees Under Water Stress)
►▼
Show Figures
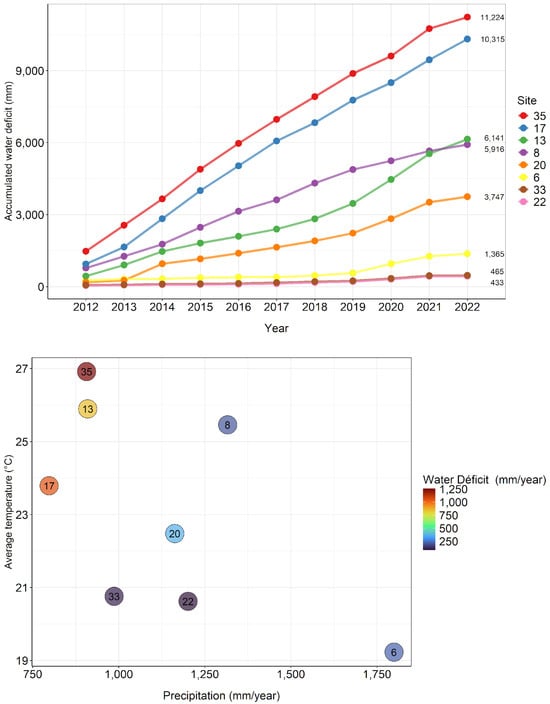
Figure 1
Figure 1
<p>Accumulated water deficit from 2012 to 2022 (<b>above</b>), precipitation, average temperature, and water deficit in the first year of the coppice rotation at the PCoppice sites (<b>below</b>).</p> Full article ">Figure 2
<p>Sprouting biomass averages at 6 months (<b>above</b>) and wood biomass averages at 12 months (<b>below</b>) of clones classified as tropical and subtropical, where n represents the number of plots of each type. The error bar indicates the standard error of the mean.</p> Full article ">Figure 3
<p>Relationships between final survival in initial rotation and initial survival in coppice rotation grouped by clone (<b>left</b>), final growth index in initial rotation, and initial growth index in coppice rotation (<b>right</b>). The black line represents the 1:1 ratio, and the blue line represents the linear regression.</p> Full article ">Figure 4
<p>Relationships between final growth index in initial rotation and initial growth index in coppice rotation at individual level by site (<b>above</b>), final uniformity (PV50) in initial rotation, and initial uniformity in coppice rotation by age (<b>below</b>). The black line represents the 1:1 ratio, and the blue line represents the linear regression.</p> Full article ">Figure 5
<p>Relationships between sprouting height at 6 months (<b>above</b>) stem biomass at 12 months (<b>below</b>), and climatic variables show that the driest sites in the initial rotation became the most productive at the beginning of the coppice rotation.</p> Full article ">Figure 6
<p>Relationship between mortality in the second rotation (R2), water deficit, and stocking.</p> Full article ">Figure 7
<p>Relationship between biomass production in the second rotation (R2), water deficit, and stocking.</p> Full article ">Figure 8
<p>Location of the 10 PCoppice project sites and the climatic types in which they are located, according to Köppen’s climatic classification. The two sites in yellow were not considered in this work. Description of the climatic types of the Koppen classification: Af (tropical rainforest climate), Am (tropical monsoon climate), As (tropical savanna climate with dry summer), Aw (tropical savanna climate with dry winter), BSh (hot semi-arid climate), Cfa (humid subtropical climate), Cfb (oceanic climate), Csa (hot-summer Mediterranean climate), Csb (warm-summer Mediterranean climate), Cwa (monsoon-influenced humid subtropical climate with dry winter), Cwb (subtropical highland climate with dry winter), Cwc (cold subtropical highland climate with dry winter).</p> Full article ">Figure 9
<p>Clonal test (<b>left</b>) and spacing test (<b>right</b>) at site 17, in Três Marias—Minas Gerais. Plots without the clone acronym have genotypes not evaluated in PCoppice.</p> Full article ">Figure 10
<p>Stump before pruning (<b>left</b>) and pruned stump (<b>right</b>) at site 8, in Inhambupe—Bahia.</p> Full article ">
<p>Accumulated water deficit from 2012 to 2022 (<b>above</b>), precipitation, average temperature, and water deficit in the first year of the coppice rotation at the PCoppice sites (<b>below</b>).</p> Full article ">Figure 2
<p>Sprouting biomass averages at 6 months (<b>above</b>) and wood biomass averages at 12 months (<b>below</b>) of clones classified as tropical and subtropical, where n represents the number of plots of each type. The error bar indicates the standard error of the mean.</p> Full article ">Figure 3
<p>Relationships between final survival in initial rotation and initial survival in coppice rotation grouped by clone (<b>left</b>), final growth index in initial rotation, and initial growth index in coppice rotation (<b>right</b>). The black line represents the 1:1 ratio, and the blue line represents the linear regression.</p> Full article ">Figure 4
<p>Relationships between final growth index in initial rotation and initial growth index in coppice rotation at individual level by site (<b>above</b>), final uniformity (PV50) in initial rotation, and initial uniformity in coppice rotation by age (<b>below</b>). The black line represents the 1:1 ratio, and the blue line represents the linear regression.</p> Full article ">Figure 5
<p>Relationships between sprouting height at 6 months (<b>above</b>) stem biomass at 12 months (<b>below</b>), and climatic variables show that the driest sites in the initial rotation became the most productive at the beginning of the coppice rotation.</p> Full article ">Figure 6
<p>Relationship between mortality in the second rotation (R2), water deficit, and stocking.</p> Full article ">Figure 7
<p>Relationship between biomass production in the second rotation (R2), water deficit, and stocking.</p> Full article ">Figure 8
<p>Location of the 10 PCoppice project sites and the climatic types in which they are located, according to Köppen’s climatic classification. The two sites in yellow were not considered in this work. Description of the climatic types of the Koppen classification: Af (tropical rainforest climate), Am (tropical monsoon climate), As (tropical savanna climate with dry summer), Aw (tropical savanna climate with dry winter), BSh (hot semi-arid climate), Cfa (humid subtropical climate), Cfb (oceanic climate), Csa (hot-summer Mediterranean climate), Csb (warm-summer Mediterranean climate), Cwa (monsoon-influenced humid subtropical climate with dry winter), Cwb (subtropical highland climate with dry winter), Cwc (cold subtropical highland climate with dry winter).</p> Full article ">Figure 9
<p>Clonal test (<b>left</b>) and spacing test (<b>right</b>) at site 17, in Três Marias—Minas Gerais. Plots without the clone acronym have genotypes not evaluated in PCoppice.</p> Full article ">Figure 10
<p>Stump before pruning (<b>left</b>) and pruned stump (<b>right</b>) at site 8, in Inhambupe—Bahia.</p> Full article ">
Journal Menu
► ▼ Journal Menu-
- Plants Home
- Aims & Scope
- Editorial Board
- Reviewer Board
- Topical Advisory Panel
- Instructions for Authors
- Special Issues
- Topics
- Sections & Collections
- Article Processing Charge
- Indexing & Archiving
- Editor’s Choice Articles
- Most Cited & Viewed
- Journal Statistics
- Journal History
- Journal Awards
- Society Collaborations
- Conferences
- Editorial Office
Journal Browser
► ▼ Journal BrowserHighly Accessed Articles
Latest Books
E-Mail Alert
News
Topics
Topic in
Agriculture, Agronomy, Crops, Foods, Plants
The Future of Farming in a Changing World: From Physiology to Technology
Topic Editors: Giuseppe Ferrara, Olaniyi Amos FawoleDeadline: 1 December 2024
Topic in
Agronomy, Horticulturae, IJPB, Life, Plants
Effects of Climate Change on Viticulture (Grape)
Topic Editors: Arif Atak, Andreia Figueiredo, Inmaculada Pascual, Fermin MoralesDeadline: 31 December 2024
Topic in
Agronomy, Crops, Forests, Horticulturae, Plants
Plants Nutrients, 2nd Volume
Topic Editors: Georgia Ntatsi, Maurizio BadianiDeadline: 31 January 2025
Topic in
Agronomy, Applied Microbiology, IJMS, Microorganisms, Plants
The XIX SEFIN Congress and 2nd Spanish-Portuguese Congress on Beneficial Plant-Microorganism Interactions (BeMiPlant)
Topic Editors: Jose Maria Vinardell, Beatriz Ramos Solano, Juan Sanjuán, Isabel V. CastroDeadline: 1 March 2025
Conferences
Special Issues
Special Issue in
Plants
Traditional Cultivars as a Genetic Source of Stress Tolerance and Quality Enhancement
Guest Editors: Marija Viljevac Vuletić, Ines MihaljevićDeadline: 25 November 2024
Special Issue in
Plants
Research on Plant Pathology and Disease Management
Guest Editors: Monika Walter, Ramon Gerardo Guevara-GonzalezDeadline: 29 November 2024
Special Issue in
Plants
Application of Remote Sensing in Crop Production and Farmland Soil Monitoring
Guest Editors: Jianjun Wang, Jiahua Zhang, Rafia Mumtaz, Minfeng XingDeadline: 29 November 2024
Special Issue in
Plants
Sustainable Weed Control Practices
Guest Editor: Andrew PriceDeadline: 29 November 2024
Topical Collections
Topical Collection in
Plants
Modeling Impacts of Changing Environmental Conditions on Plant Growth
Collection Editor: Dominik Schmidt
Topical Collection in
Plants
Feature paper in Plant Response to Abiotic Stress and Climate Change
Collection Editors: Veronica De Micco, Luigi Sanita' di Toppi
Topical Collection in
Plants
Advances in Plant Breeding
Collection Editors: Radu E. Sestras, Jaime Prohens, Adriana F. Sestras, Mariola Plazas
Topical Collection in
Plants
Selected Papers from Lithuanian Research Centre for Agriculture and Forestry
Collection Editors: Giedrė Samuolienė, Gražina Kadžienė, Darius Kviklys, Neringa Rasiukeviciute